Abstract
The “Greenhouse Earth” of the late Paleocene and early Eocene was generally characterized by warm temperatures and elevated Partial Pressure of Carbon Dioxide (pCO2). During this time, however, at least two “hyperthermals” occurred, the most pronounced corresponding to the Paleocene/Eocene Boundary ca. 55 million years ago (Ma). These were geologically brief (<200 kyr) events that began with rapid warming across the globe and massive input of 13C-depleted carbon to the ocean and atmosphere. They were also times of extreme variations in ecosystems and the hydrological cycle. Although cause and effect relationships during hyperthermals, as well as links between them, remain uncertain, they are essential intervals in which to study and discuss global warming and climate change.
1. Introduction
“Scientific Basis for Climate Change”, published by the Intergovernmental Panel on Climate Change (IPCC) in 2007 (http://www.ipcc.ch/ipccreports/ar4-wg1.htm), has become the authoritative body of work in which to frame science and policy regarding greenhouse gas emissions and global climate change. The vast majority of this 996-page document (~1100 pages including Supplementary Information) pertains to theory, recent observations, and potential consequences. Although it includes a chapter on past climates, less than 4 pages are devoted to Earth before the Quaternary. This is remarkable, especially given our current understanding of the early Paleogene.
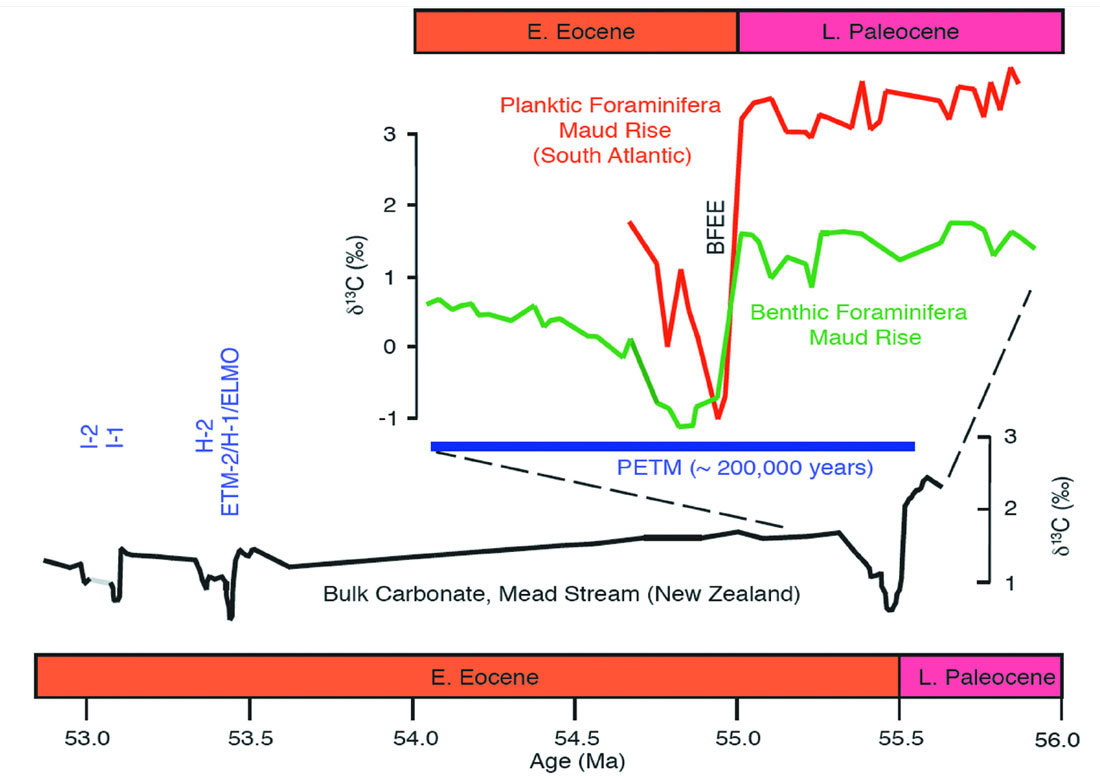
Although absolute temperatures and latitudinal temperature gradients remain difficult to constrain, Earth warmed by ~5 °C between 58 and 50 Ma (Zachos et al., 2008). By the end, mean annual temperatures at polar latitudes were 12-18°C higher than today, an assertion consistently supported from the fossil record (e.g., reptile assemblages on Ellesmere Island; palm fronds in Wyoming). Superimposed on this overall warming trend, however, were at least two events characterized by rapid additional warming and massive carbon input (Figure 1). These events are the Paleocene-Eocene thermal maximum (PETM) and Eocene thermal maximum 2 (ETM-2). Here I introduce these and related events, highlight their significance to understanding global climate change, examine several predictions for Earth’s future within the context of past observations, and present current overarching problems. Except for three key references, the emphasis is on work published in the last four years.
2. The PETM and Early Eocene Hyperthermals
For over 100 years, paleontologists have recognized significant biotic turnovers near the transition from the Paleocene to Eocene. These include a mass extinction of benthic foraminifera in deep ocean sites and profound animal and plant migrations in northern hemisphere locations. However, the interval moved beyond the focus of stratigraphy and biotic evolution with two seminal papers. Kennett and Stott (1991) examined sediment deposited on Maud Rise in the southern Atlantic Ocean; they demonstrated that the benthic foraminifera extinction precisely corresponded to pronounced negative excursions in the oxygen isotope and carbon isotope compositions (δ18O and δ13C) of carbonate tests made by planktic foraminifera and benthic foraminifera (Figure 1). Koch and colleagues (1992) examined sequences in the Bighorn Basin of Wyoming; they showed that the abrupt turnover of mammals (i.e., the Paleocene/Eocene boundary) precisely corresponded to a pronounced negative excursion in the δ13C of soil carbonate and mammalian tooth enamel. Together, the papers suggested major simultaneous changes in marine and terrestrial environments that were somehow linked to Earth surface warming (the decrease in δ18O) and a fundamental change in the global carbon cycle. From sedimentation rates at the studied locations, it was also clear that the entire event, from onset through recovery, must have occurred within < 200,000 years (Figure 1).
The Paleocene/Eocene transition has since become the focus of hundreds of papers (Sluijs et al., 2007a), and has received a commonly used name (PETM) and an approximate age (55.5 Ma). In fact, the start of the PETM now marks the Paleocene/Eocene boundary. Good summaries of the event are provided in recent articles (Bowen et al., 2006; Sluijs et al., 2007a; Zachos et al., 2008). However, to appreciate the PETM, it is useful to examine records at specific locations.
Integrated Ocean Drilling Program (IODP) Expedition 302 drilled a series of holes at ~87.5°N latitude on Lomonosov Ridge in the central Arctic Ocean. The ridge represents a fragment of continental crust that rifted from northern Eurasia in the Paleocene and subsequently subsided, although with minimal change in latitude. The recovered Cenozoic section extends, albeit discontinuously, from the seafloor to ~ 410 m below seafloor (mbsf). Within this record is an intriguing 4-5 m thick interval between 381-386 mbsf that stands out visually because portions are laminated (Sluijs et al., 2006). However, it is analyses of the enclosed sediment that show it to be special (Figure 2). The δ13C of bulk organic carbon and n-alkanes display -6‰ negative excursions within 1-2 m followed by low values and a recovery to near initial values afterwards; within this horizon, subtropical dinoflagellates suddenly appear and crenarchaeotal lipid concentrations suggest a 6°C warming to temperatures of ~23°C (Sluijs et al., 2006; Pagani et al., 2006b). These temperatures may reflect summer or mean annual conditions but are extreme for the location nonetheless. Among the dinoflagellate cysts are Apectodinium Augustum, a species to date only found during the PETM. Given average Palaeogene sedimentation rates of 17 m/Myr at the site, the interval was seemingly deposited within 200,000 to 300,000 yrs.
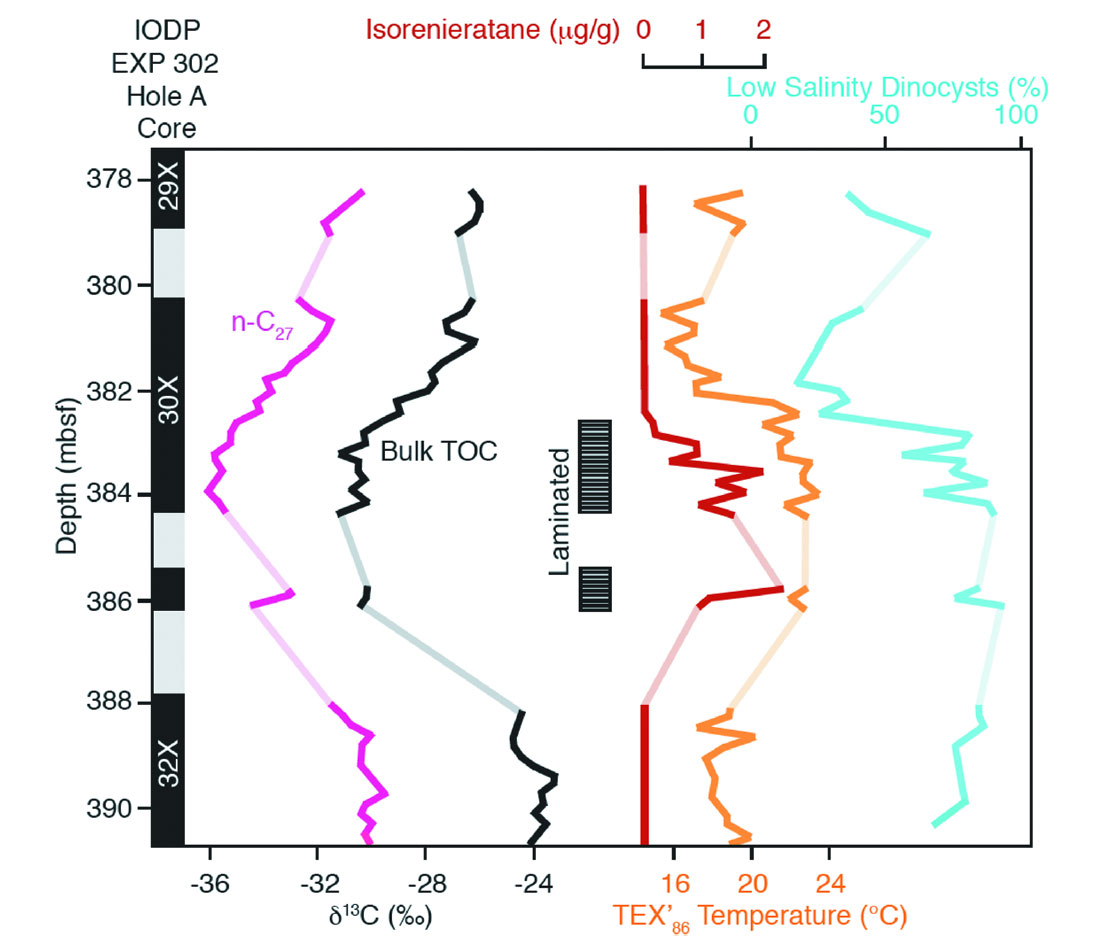
While unusual considering its exotic location near the North Pole, the PETM recovered at Lomonosov Ridge serves as a template for the event as expressed in numerous sedimentary records worldwide. Within a short interval, there were profound changes in environmental conditions and biota. These began with significant global warming (around 6°C), and a remarkable global drop in the δ13C of all primary carbon-bearing components of sediment (e.g., Sluijs et al., 2007a). Typically, the event is expressed by a lithological change—laminations in the central Arctic but color changes, clay-rich horizons, conglomerates and other facies elsewhere (e.g., Zachos et al., 2005; Schmitz and Pujalte, 2007; Nicolo et al., 2007; Sluijs et al., 2008). The event occurred within <200,000 years on the basis of sedimentation rates, cyclostratigraphy and He-isotope accumulation. Much of the overall duration involved the recovery, so that the changes initiated within <50 kyr (e.g., Sluijs et al., 2007a).
The PETM has been called a “hyperthermal” in recent papers (e.g., Nicolo et al., 2007; Sluijs et al., 2008). For lack of a formal definition, this is referred to as a geologically brief interval of extreme global warming and environmental change. Outstanding issues are whether other hyperthermals exist and whether they have characteristics similar to the PETM. Recent studies suggest that a series of brief events punctuate the early Eocene (Figure 1; Lourens et al., 2005; Nicolo et al., 2007; Sluijs et al., 2008). The first of the post-PETM events, ETM-2 (also known as H-1 or ELMO) has been shown to coincide with earth surface warming (Lourens et al., 2005). There was also an event in the early Toarcian, ca. 183 Ma, which has characteristics similar to those of the PETM (Cohen et al., 2007).
3. Significance of the Early Paleogene Events
As noted above, the PETM was characterized by a decrease in the δ13C of carbon across Earth’s surface (Figures 1, 2). The magnitude of this carbon isotope excursion (CIE) varies depending on the location, the carbon phase analyzed, and the completeness of the sedimentary record (Bowen et al., 2006; Sluijs et al., 2007a). Where the entire PETM is preserved, the CIE is typically -2 to -3 ‰ in bulk carbonate and benthic foraminifera. However, it is often -4 to -6 ‰ in organic carbon (including specific compounds such as nalkanes), planktic foraminifera and soil carbonate. The larger magnitudes can be explained, at least in some cases, by changes in environmental conditions during the PETM. For example, the -6 ‰ CIE in bulk organic carbon and n-alkanes across the PETM on Lomonosov Ridge partly reflects a decrease in the abundance of gymnosperms relative to angiosperms on nearby continents (Schouten et al., 2007). Large magnitude δ13C excursions in planktic foraminifera (e.g., Handley et al., 2008) may in part reflect vertical migration of calcifying organisms to deeper and colder water. In other words, the CIE recorded in individual records represents a global signature modified by local effects, a fact that has been obvious from early work (Kennett and Stott, 1991; Koch et al., 1992).
A truly global CIE implies a change in carbon inputs or outputs to the exogenic carbon cycle. This cycle comprises all carbon in the ocean, biosphere and atmosphere. Because carbon cycles between these reservoirs fairly quickly (< 2000 years at presentday), a variation in the δ13C of carbon fluxes will impact all reservoirs. Given the geologically short duration of the PETM and that the event involved global warming, its CIE was attributed about 15 years ago to a massive CO2 input (Dickens et al., 1995). This notion, somewhat controversial at the time, has become less so with subsequent work, as also emphasized below.
The significance of a rapid and global -2 to -3 ‰ CIE caused by a CO2 injection is partly appreciated through straightforward mass balance calculations (Dickens et al., 1995). The present-day exogenic carbon cycle contains about 40,000 gigatonnes (Gt = 1015 g) of carbon with an average δ13C of -1.45 (the value is negative because the total includes 13C-depleted organic carbon). To change this enormous mass by -2 to -3 ‰ necessitates a tremendous addition of carbon, the amount depending on the duration and the isotopic composition of the carbon input (Figure 3). For the present-day exogenic carbon cycle, a 10,000 yr injection of 2000-3000 Gt of carbon with a δ13C of -60 ‰ would cause a -2 to -3 ‰ CIE. This theoretical input would increase with a longer duration, a less 13C-depleted source, or a greater amount of carbon within the exogenic carbon cycle. The CIE at the PETM signifies that at least 2000-3000 Gt of carbon rapidly entered the ocean, atmosphere and biosphere.
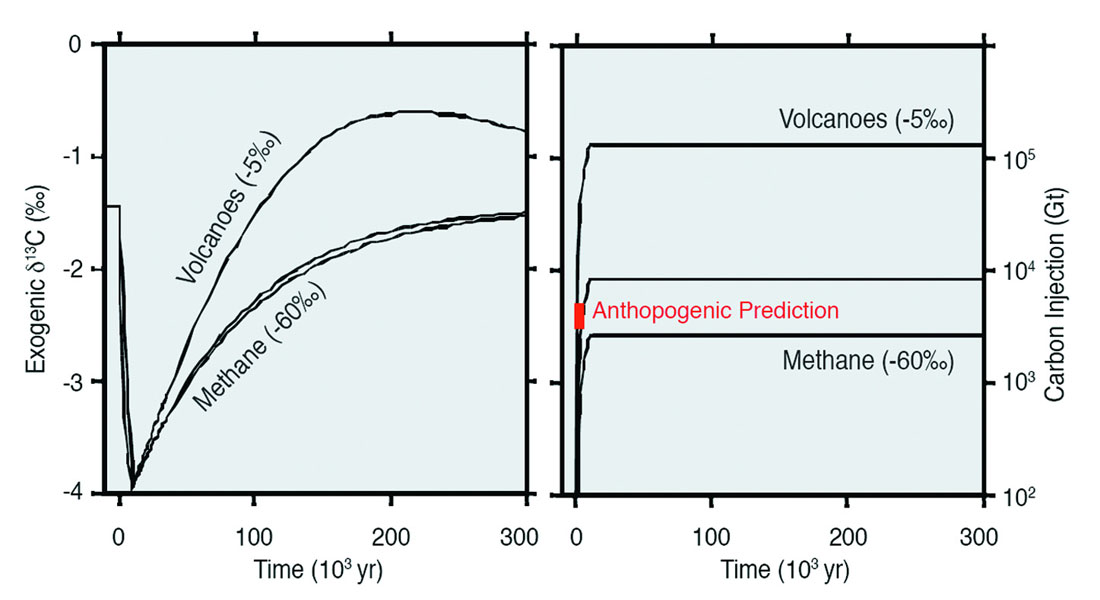
The full import of the PETM to studies of global climate change is realized by a comparison to anthropogenic carbon emissions. Our society presently adds about 8Gt of 13C-depleted carbon to the exogenic carbon cycle each year. By the year 2500, the cumulative injection will likely be between 3000 and 4000 Gt (Figure 3). While the present rate of carbon addition is faster than what happened during the PETM, the magnitude and isotopic signature are similar. Assuming ETM-2 and other early Eocene were causally related to the PETM, there exists a series of past analogs in which to assess the impact of massive carbon release to the exogenic carbon cycle.
4. Environmental Consequences of Massive Carbon Addition
“Scientific Basis for Climate Change” presents numerous potential outcomes resulting from anthropogenic CO2 addition to the atmosphere. Global warming has been widely discussed in the literature and media, and definitely occurred during the PETM. As noted in the following section, though, cause and effect relationships between carbon input and temperature are not clear during the event. Nonetheless, four other “very likely” outcomes of massive CO2 addition or elevated temperature appear to have happened during the PETM.
Rapid input of CO2 to the atmosphere (or ocean) should decrease the pH of the ocean. An obvious geological consequence of this “ocean acidification” would be carbonate dissolution. Although the IPCC document focuses mostly on surface waters and the impact on marine calcifying organisms, the more pronounced effect on the >1000-yr time scale would be rises in the lysocline and the calcite compensation depth (CCD). These are depth horizons in the deep ocean delineating the onset of significant carbonate dissolution and the termination of carbonate accumulation, respectively. Numerous early Paleogene sections deposited in deep-sea environments show a prominent carbonate dissolution horizon across the PETM (e.g., Zeebe and Zachos, 2007). Perhaps the best existing example of this phenomenon comes from drill sites down the flanks of Walvis Ridge in the southern Atlantic Ocean. Sections recovered at these sites demonstrate a progressive and rapid shoaling of the CCD at the start of the PETM (Zachos et al., 2005). It is emphasized here that widespread dissolution of carbonate in deep-sea environments across the PETM necessarily indicates that the CIE resulted from massive input of CO2 to the ocean or atmosphere. Recent work suggests that seafloor carbonate dissolution also characterizes ETM-2 and other events in the Early Eocene marked by negative δ13C excursions (e.g., Lourens et al., 2005; Nicolo et al., 2007).
Elevated temperatures should raise sea level because of thermal expansion of water and because of melting of continental ice. Although the Early Paleogene probably had much less ice than today, a eustatic rise occurred across the PETM. Outside of southeast Europe, the PETM in shallow marine sections generally contains evidence for increased distance to the coast (Sluijs et al., 2008). This includes shifts in dinoflagellate cysts to more marine assemblages and increases in the proportion of marine organic carbon. The most compelling argument for a eustatic rise, however, comes from sections that were deposited on land but proximal to the coast before the PETM. At these sites such as at Kumara, New Zealand, terrestrial sediments sandwich an anomalous marine unit that marks the PETM (Sluijs et al., 2008).
Climate models consistently indicate that annual precipitation in the Arctic region will increase significantly with higher temperatures. This is because of an accelerated hydrological cycle that carries more water to high latitudes. The laminated PETM section recovered from Lomonosov Ridge (Figure 2) suggests that the Arctic Ocean became stratified and that dissolved oxygen did not reach the seafloor during the event. This interpretation is supported by analyses of organic carbon, which contain isorenieratane, an unusual compound produced by bacteria that inhabit the photic zone of stratified water bodies such as the modern Black Sea (Sluijs et al., 2006). Dinoflagellate cyst assemblages further indicate that stratification resulted from freshening of surface water above the location despite a greater distance to the coast (Sluijs et al., 2006). Given the rises in temperature and sea level, the simplest explanation is that large volumes of fresh water discharged into the Arctic Ocean during the PETM. A large positive excursion in the hydrogen isotopic composition of n-alkanes across the PETM at Lomonosov Ridge may, in fact, indicate greater moisture transport to high latitudes (Pagani et al., 2006b).
A fascinating result of climate simulations at elevated atmospheric pCO2 concerns precipitation extremes and droughts. For many locations, rapid and significant global warming should increase annual precipitation but concentrate it within a shorter duration. Effectively, throughout a year, wet seasons become more intense while dry seasons become longer. This should enhance the erosion and discharge of sediment given observations of modern environments (e.g., Schmitz and Pujalte, 2007). Such an effect probably occurred during the PETM. In northern Spain, the event precisely coincides with a widespread and prominent conglomerate interpreted as a “megafan” (Schmitz and Pujalte, 2007). Asudden increase in siliciclastic accumulation also marks the PETM in several continental margin sequences despite the rise in sea level (Nicolo et al., 2007; Sluijs et al., 2008). A similar response along continental margins appears to characterize other suspected early Eocene hyperthermals (Nicolo et al., 2007; Sluijs et al., 2008).
5. Summary and Current Problems
There is now widespread consensus in the scientific community that the PETM represents a geologically brief interval of global warming and massive carbon input (Zachos et al, 2008). An emerging view is that the event represents the extreme case of a recurring phenomenon in the geological record (e.g., Nicolo et al., 2007, Cohen et al., 2007). Increasingly, and for good reason, there is a tendency to evaluate observed variations across the PETM in terms of predictions for future environmental change (e.g., Bowen et al., 2006; Zachos et al., 2008). With available information, it is fair to say that certain expectations from global climate models are qualitatively consistent with occurrences during the PETM and related events. That stated there are some fundamental problems.One outstanding issue is the mass of carbon injected during the PETM. Theoretically, the size of a carbon addition can be determined from the magnitude of the CIE and the amount of carbonate dissolved on the seafloor (Dickens et al., 1995; Figure 3). Both are open to debate (Pagani et al., 2006b; Handley et al., 2008). For example, drilling on Walvis Ridge demonstrated that the CCD shoaled by at least 2 km during the PETM at this location (Zachos et al., 2005). Some authors (e.g., Zachos et al., 2005; Pagani et al., 2006a) have taken this as representative of the entire ocean, which would necessitate a carbon input far exceeding 2000 Gt. Sediment records from the much larger Pacific Ocean basin, however, indicate a much smaller rise in the CCD (Zeebe and Zachos, 2007). As previously noted for δ13C records, local effects (such as a change in water properties above Walvis Ridge) probably modify signals across the event. An input of 2000 Gt should be viewed as a minimum estimate to explain basic observations during the PETM.
A related and crucial issue concerns climate sensitivity and the observed 6°C warming during the PETM. The relationship between atmospheric pCO2 and earth surface temperature is not straightforward because of numerous feedbacks. Nonetheless, it is convenient to consider the temperature response to a doubling of pCO2. Atmospheric pCO2 in the latest Paleocene (i.e., before the PETM) was probably between 1000 and 2000 ppmv (Zachos et al., 2008). A 2000 Gt input of carbon to the exogenic carbon cycle cannot explain the 6°C warming, unless earth surfaces temperatures increase by more than 5°C per doubling of pCO2 (Pagani et al., 2006a). Such climate sensitivity is more extreme than that in most climate models. Complicating matters, however, is the relative timing of environmental change and massive carbon addition at the start of the PETM. In several sediment sequences, changes in temperature and biota begin before the start of the CIE (Sluijs et al., 2007b). With available data, massive carbon addition during the PETM appears to have been a positive feedback to environmental change initiated by some process that remains highly speculative.
Perhaps the most confounding problem with the PETM is the source of the carbon injection. Even the low estimates of 2000- 3000 Gt are tremendous considering the sizes of carbon reservoirs on the pre-industrial Earth. For example, the entire terrestrial biosphere, including soil and humus, contained about 2000 Gt, and worldwide conventional fossil fuel resources were about 5000 Gt. Within this context, there are only a few plausible sources, and mass balance calculations seem to preclude volcanic CO2, which is too enriched in 13C, and the biosphere, which is not sufficiently large (Dickens et al., 1995). Moreover, some suggestions, such as the impact of a carbonaceous comet or subaerial exposure of marine carbon are inconsistent with basic observations (Kopp et al., 2007; Lippert and Zachos, 2007; Sluijs et al., 2008). Possible sources discussed in much of the above literature are thermal dissociation of gas hydrates in marine sediment, burning of extensive peat deposits and contact metamorphism of a large petroleum system in the northern Atlantic Ocean.
Join the Conversation
Interested in starting, or contributing to a conversation about an article or issue of the RECORDER? Join our CSEG LinkedIn Group.
Share This Article