Introduction
The availability and fidelity of broadband seismic instruments in recent years have greatly accelerated worldwide research on ground motion and seismic structure. Equipped with instruments that are capable of digitally recording signals across a wide frequency band (0.01-100 Hz), earthquake seismologists no longer need to contend with analogue records, limited data density or inaccurate readings from microfiche machines. Spearheaded by the Incorporated Research Institutions for Seismology (IRIS), founded in 1984, hundreds of gigabytes of high-quality digital earthquake data from permanent global arrays and temporary deployments can now be disseminated over the Internet every day for near-real-time processing and analysis. The exceptional data quality and coverage, highlighted by USArray (USA), Hi-net/J-Array (Japan) and Yellowknife Array (Canada) have overcome many conceptual and practical barriers between exploration and global seismology. As a direct result, the ‘global community’ can now take advantage of high-resolution, often exploration-based, methods that are predicated upon superior data quality to monitor/characterize seismic sources and structure. In other words, the emergence of broadband seismic arrays has narrowed the gap between controlled source surveys and regional/global seismology considerably.
In this paper we present an overview of the establishment of the Canadian Rockies and Alberta Network (nicknamed CRANE), the first semi-permanent broadband seismic array in Alberta, and some of the results from the first two years of operation. We highlight ongoing research that improves our ability to interpret seismic signals both from the near-surface (e.g., lakewave induced) and from tens or hundreds of kilometers beneath our feet. While some of the results are still preliminary, most of the documented efforts have shown significant promise in understanding the ground motion, tectonic history and dynamics in our region.
Array Setup and Seismic Data Acquisition
The field deployment began in late 2005 under the funding support of the Canadian Foundation for Innovation (CFI) and the University of Alberta (from here on, UofA). Six broadband seismic instruments were initially acquired and installed in central and southern Alberta, mostly on private land relatively removed from cultural and industrial noise. The array has since been expanded to 11 stations and presently forms a semi-uniform grid with an average station spacing of ~150 km (Figure 1). Most of the CRANE stations are equipped with three-component (east-west, north-south and vertical) Trillium 240 sensors (manufactured by Nanometrics Inc.) that exhibit a relatively flat response curve between the corner frequencies of 0.005 Hz (low) and 100 Hz (high) (Figure 2a). Each instrument is connected to a digitizer sampling at 20 Hz. The sensor and digitizer are housed within two separate underground vaults, powered by a 30-80 W solar panel and a 12V rechargeable battery (Figure 2b); see Figure 2c for a schematic diagram of this self-sustaining system. These vaults are sealed and well insulated to 1) protect against water or animal-related damages, 2) reduce instrument noise associated with severe diurnal temperature changes, and 3) ensure proper functionality during the harshest winter months. Despite extensive efforts in site selection and installation, the deployment did not go without a hitch: one of the original sites (REC) had to be relocated after ~8 months due to an unexpected level of ground distortion surrounding the vaults, most likely due to frost heave.
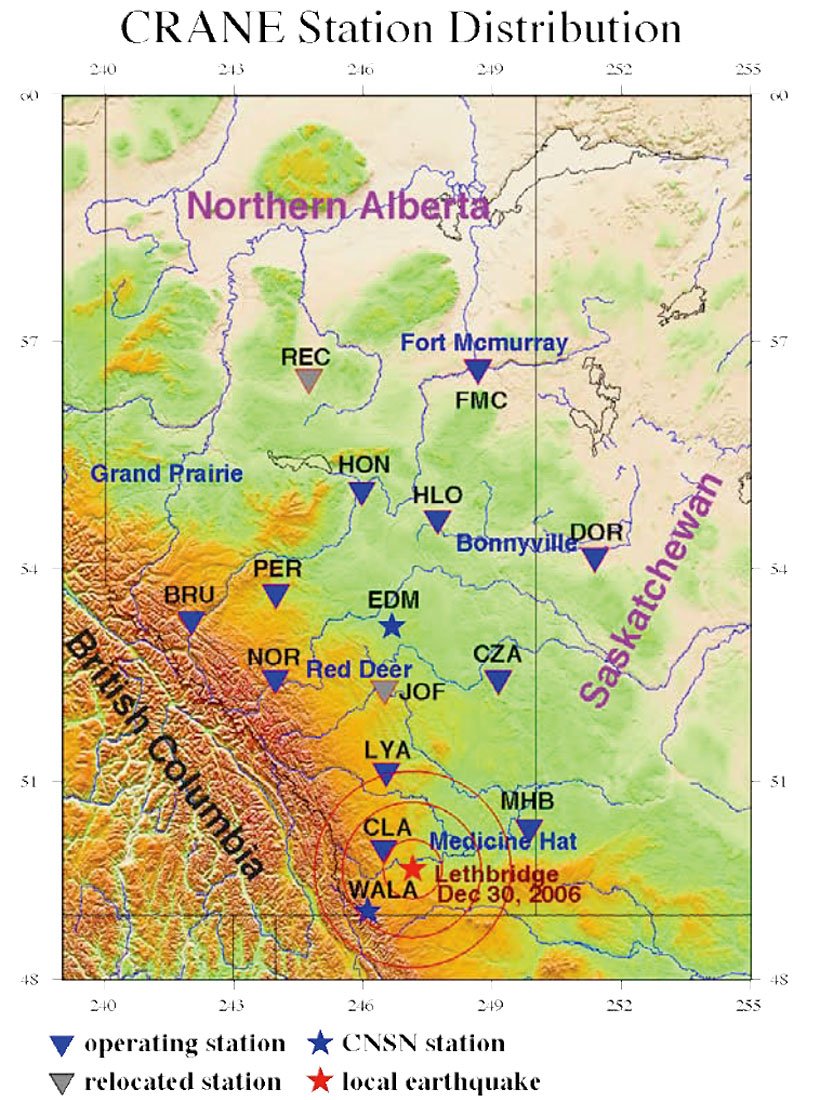
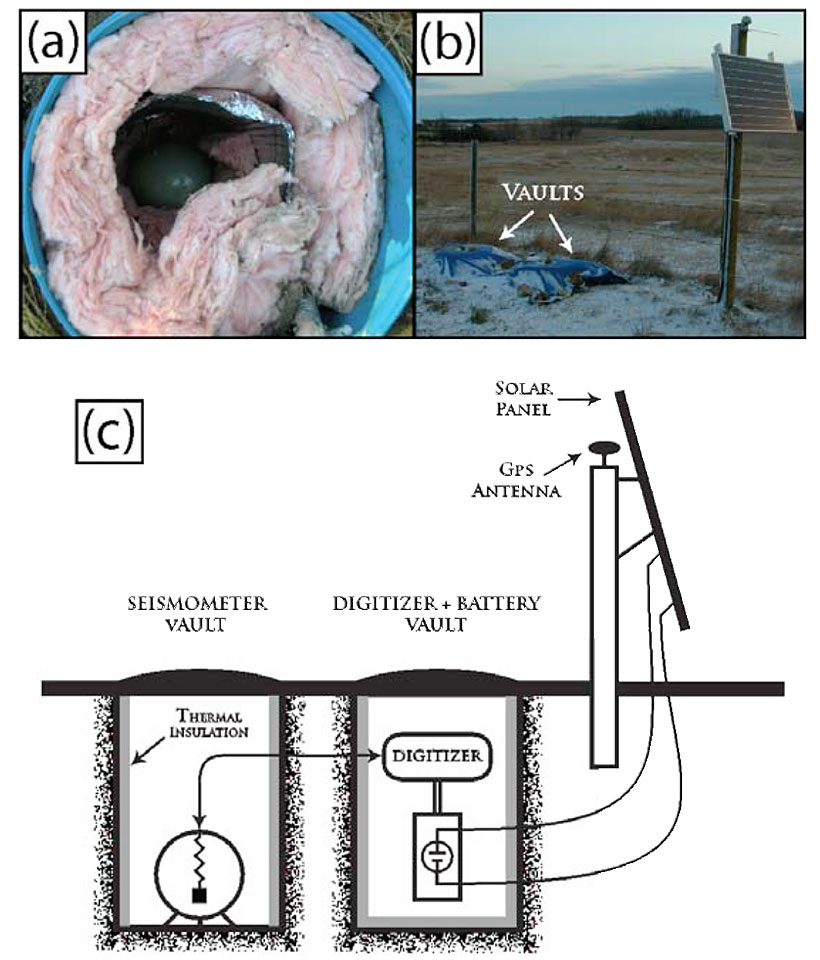
The majority of the CRANE stations have been operating continuously since early 2007, recording more than 300 Mw>5 earthquakes over the past 2+ years. Figure 3 shows sample waveforms from a devastating Mw 7.9 earthquake (Sichuan, May 2008) and a modest Mw 5.0 earthquake in Alaska (Apr 2009). In both cases, P, S and surface wave arrivals are consistently identified on the vertical-component seismograms; the quality of the vertical component at most of the CRANE stations is superior to that of the horizontal components. A comprehensive database has been developed at the UofA for easy access and analysis of the teleseismic waveforms and travel times. The availability of global earthquake signals provides a valuable resource and a rare opportunity to decipher the lithosphere/mantle structure beneath the Alberta portion of the Western Canadian Sedimentary Basin (WCSB).
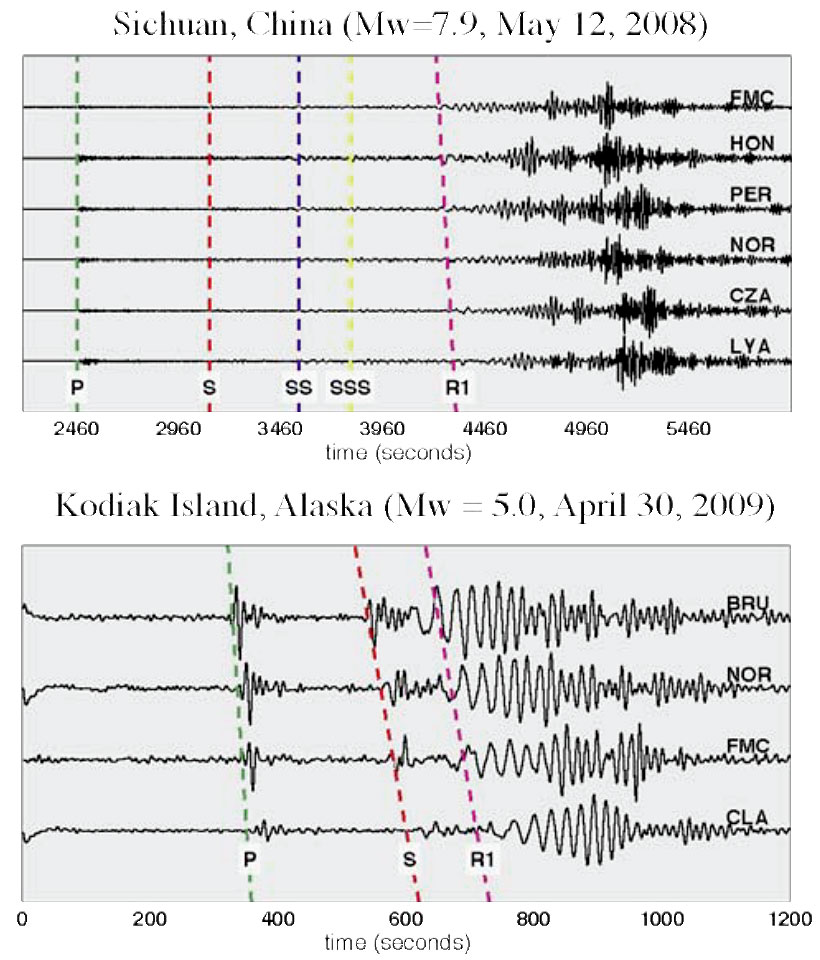
In addition to teleseismic data, the CRANE array has successfully recorded several smaller-magnitude (Mw < 4.5) earthquakes from Alberta and surrounding regions including British Columbia, Washington, Oregon and Montana. Figure 4 shows the unfiltered vertical-component recordings from an Mw 3.5 earthquake near Lethbridge, Alberta. Strong highfrequency (>1 Hz) signals are observed at CLA and LYA, two stations that are less than 300 km away from the epicenter. The timing of the P wave arrivals, which roughly correlates with epicentral distance, is highly sensitive to the crustal structures sampled by the ray paths. Severe amplitude decay is visible at longer distances, which indicates strong attenuation within the sedimentary cover of the WCSB.
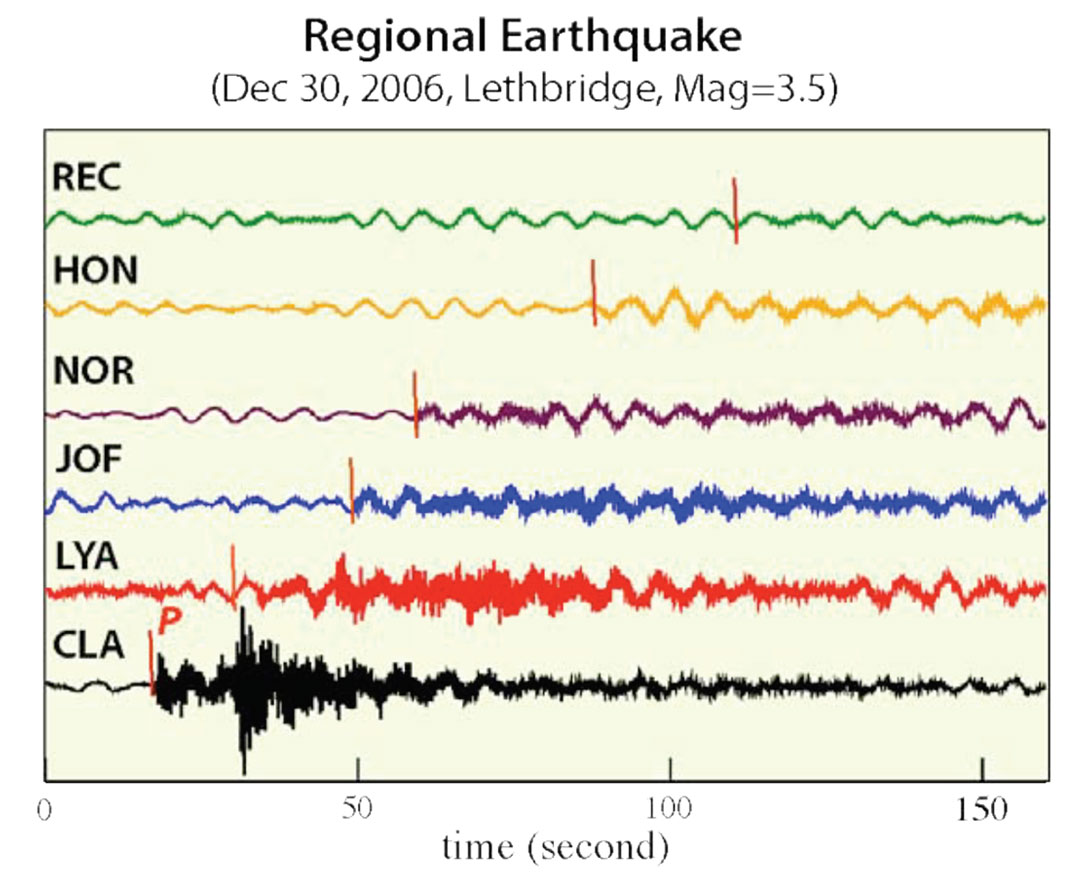
The data analysis phase of the infrastructure project is presently underway. Ongoing efforts emphasize the investigation of seismic sources and structure using both the conventional teleseismic body/surface waves and the ‘noisy’ (or, uneventful) part of a seismic record. A key objective is to integrate proven methodologies with novel and promising approaches. Considerable progress has been made in understanding regional seismic structure, local earthquakes, microseisms and anisotropy. For brevity we only present key research results on regional microseisms, which are sensitive to surface processes and crustal structure, and shear-wave splitting measurements that constrain seismic anisotropy.
Source and Structure Imaging Using Microseismic Signals
Since the late 1990s, techniques based on the cross-correlation of ambient noise fields have been overwhelmingly successful for a broad spectrum of problems in physics, geophysics (both global and exploration-based), engineering, acoustics and medical imaging (see reviews by Weaver, 2005; Snieder et al., 2009). The data and application vary substantially among the various disciplines, but the vast majority of the studies aim to complete one or both of the following tasks: 1) to extract the Green’s function between two receivers by cross-correlating their noise records (Clarebout, 1968), and 2) to locate and characterize the source of dominant noise signals (e.g., Sabra et al., 2005; Gu et al., 2007; Yang and Ritswoller, 2008). When the noise sources are uniformly distributed (or, ‘ambient’), it can be mathematically proven that the cross-correlation (or its derivative) of noise records at two nearby receivers is symmetrical about the zero lag time and equivalent to the Green’s function (Snieder, 2004). This result forms the basis for an increasing number of high-resolution, noise-based images of regional seismic structure; successful examples include California (e.g., Shapiro et al., 2005; Sabra et al., 2005), southern China (Yao et al., 2006) and Europe (Yang et al., 2006; Brzak et al., 2009). Symmetric noise correlation functions from CRANE array have recently been included in the tomographic inversion for the seismic structure beneath central Alberta. However, the existence of dominant/non-ambient noise sources presents a major element of uncertainty. For instance, strong tides can directly affect the accuracy of structure imaging based upon noise correlations.
Persistent (both ambient and non-uniform) regional sources have been identified from the CRANE data. This task involves processing and normalizing (Bensen et al., 2007) a full year of continuous records for each station, and then correlating the waveforms at all available frequencies (Brzak et al., 2009). The vertical-component correlation functions from all station pairs, irrespective of station azimuths, exhibit distance-time moveouts that are consistent with Rayleigh waves propagating from one station to another (Figure 5a). For the selected frequency range (0.03-0.06Hz), the delay (lag) times of the correlation peaks accurately reflect the Rayleigh wave phase velocity between the respective station pairs. The subsequent dispersion analysis shows strong asymmetry for a number of station pairs in the frequency range (0.08, 0.15 Hz) (Figure 5b). The lack of coherent moveout on either side of the zero lag is clear evidence of persistent regional or global ‘noise’ sources (e.g., Schulte-Pelkum et al., 2004; Gu et al., 2007).
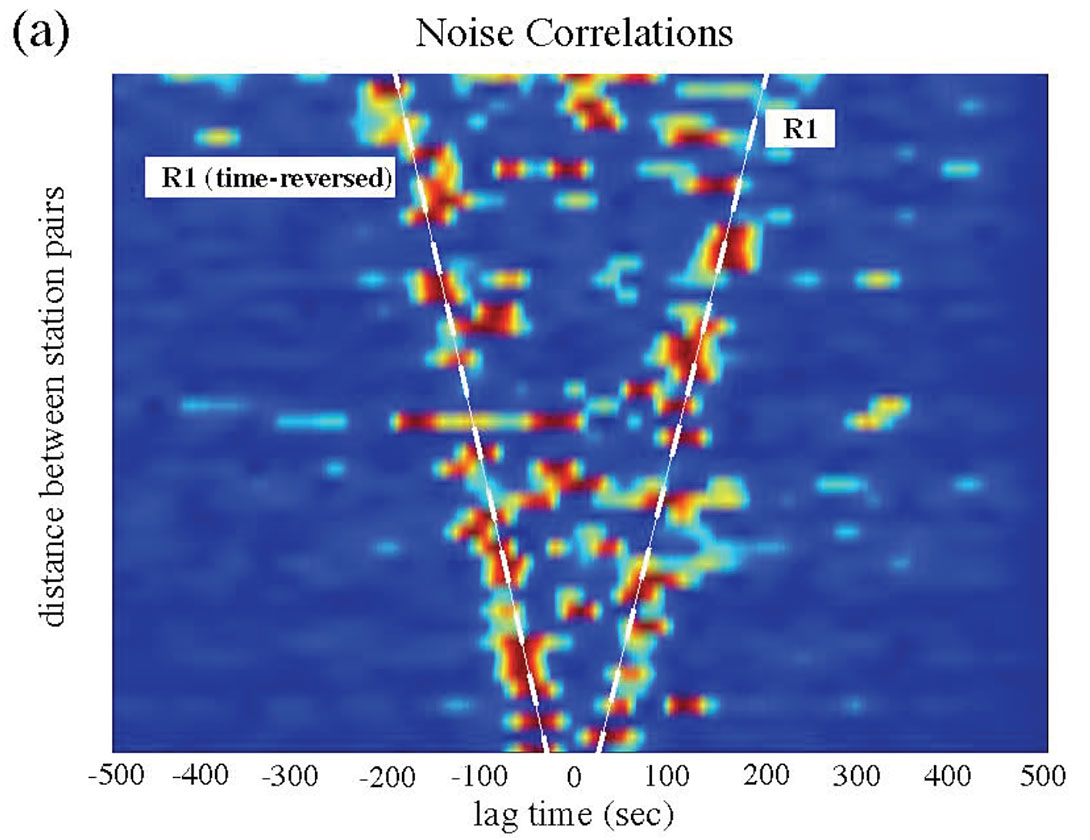
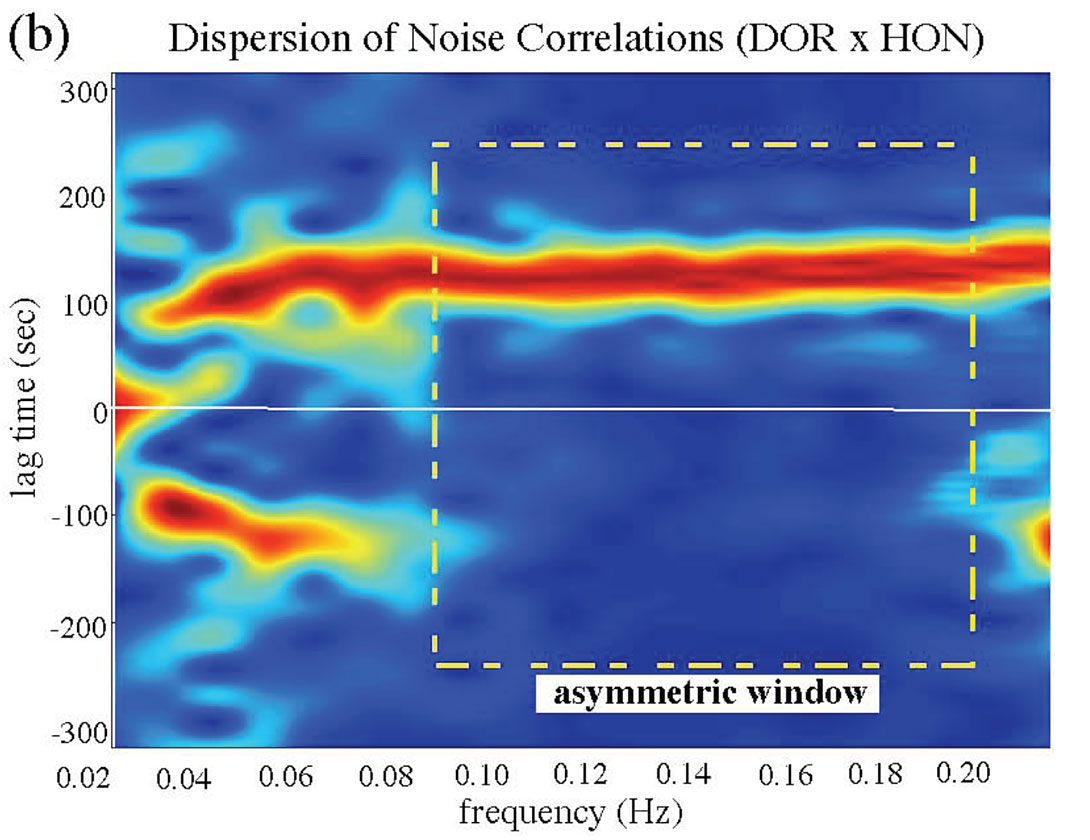
Asymmetric noise sources at intermediate period range (5-20s) have widely been attributed to ground motions caused by ocean swells and/or coastal impact (Longuet-Higgins, 1950). These modest but persistent sources generally exhibit strong directivity and, in some regions, seasonal variations (e.g., Schulte-Pelkum et al., 2004; Yang and Ritzwoller, 2008; Brzak et al., 2009). For the CRANE array, the positions/signs of the correlation peaks relative to zero lag time are, to first order, consistent with the expected microseisms from the Pacific ocean. The optimal source position is located in the western Pacific Ocean (near the Tonga- Kermadec region) based on a waveform migration algorithm (Brzak et al., 2009; Gu and Shen, in preparation, 2009). Its substantial distance from the east coast suggests an origin likely associated with the nonlinear interaction of oceanic waves. Similar global noise sources have recently been documented in the vicinity (Yang and Ritswoller, 2008).
A second, more interesting finding is a subtle noise source near station HON (Figure 6a). This source is highly non-ambient (see Figure 5b), fanning out to both the CRANE stations and permanent stations operated by the Canadian National Seismic Network (CNSN). The square of the correlation functions are shown in Figure 6b, where the negative arrival times of the correlation peaks indicate time delay of propagating Rayleigh waves relative to station HON. In almost all cases, the negative peaks follow a consistent distance-time relationship starting from station HON and dwarf their positive (time-reversed) counterparts.
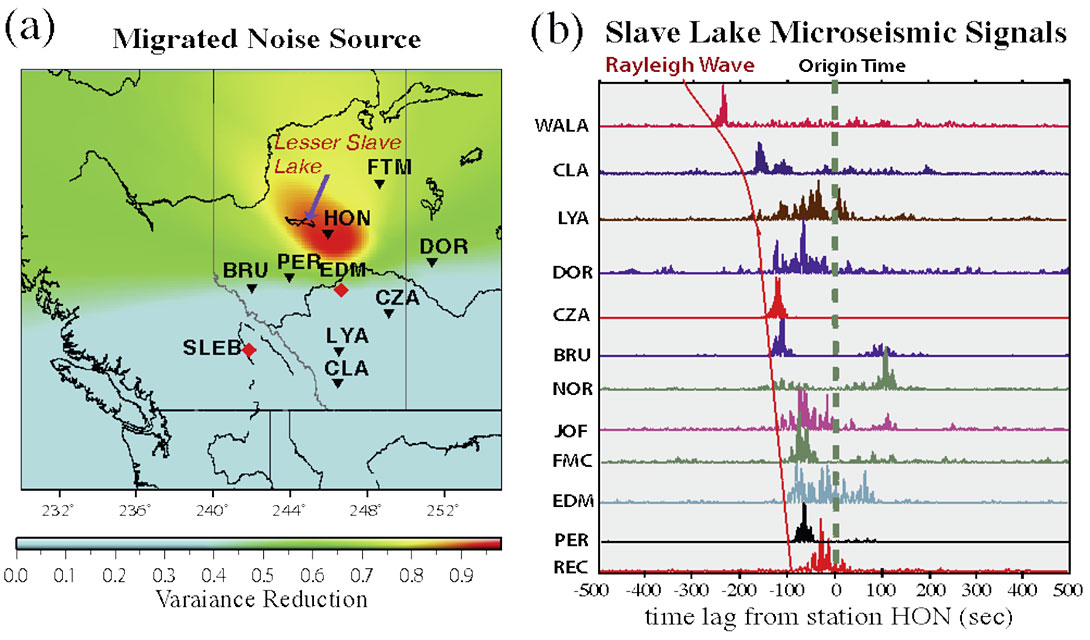
The anomalous noise northwest of the CRANE array, covering mainly the Lesser Slave Lake region, cannot be attributed to industrial (oil/gas survey or logging) noise. Such activities are capable of producing detectable signals on nearby broadband sensors, but their dominant frequency ranges (>5 Hz) are significantly higher than the observed range from 0.08 Hz to 0.15 Hz. Furthermore, while considerable oil drilling took place in the Lesser Slave Lake region during the CRANE deployment, the extensive oil/gas activities in other parts of the province and the lack of corresponding noise sources is difficult to reconcile.
The most probable source of the dominant local noise is water waves, especially in the well documented ‘ocean microseism’ frequencies range of 0.1-0.2 Hz (Longuet-Higgins, 1950). While Alberta basin is devoid of oceans or seas, the potential source location overlaps with large water bodies including Lesser Slave Lake and parts of Athabasca River (the limited number of station pairs prevents a more precise source location). Effects of rivers can be further eliminated as a candidate based on two observations. First, while rivers have been previously documented as a source of microseisms (Birtin et al., 2008), the discharge and topographic relief (which aids the water impact) near Lesser Slave Lake pale in comparison to those near the Rocky Mountain foothills where the river originates. However, we do not detect microseismic sources in the vicinity of the Rocky Mountain. Seasonality analyses via independent examination of the noise signals for the summer (May 2008-Oct 2008) and winter (Dec 2007-March 2008) months suggest that the noise source occurred during the latter time range. Near-ambient, symmetric noise signals from the summer months are strong evidence against the effect of open waters, including that from the Lesser Slave Lake during the summer time.
The sources of the observed microseismic signals from the Lesser Slave Lake region remain debatable. However, as the largest lake in Alberta and one of the Canada’s most popular ice fishing sites, constant traffic on the frozen lake (via various ice roads, starting near December each year) can cause significant vibrations of the lake ice layer. These vibrations, which are easily felt while standing on the ice surface, can be transmitted to the lake bottom and shore through the water layer. The frozen lake, which spans over 1100 square kilometers, acts as a filter and only certain frequencies (e.g., 0.06-0.2 Hz) are retained, resonated and potentially amplified. Increased traffic surrounding the lake during the winter months may also contribute to episodic pressure changes within the lake.
Our study represents one of the first reports of lake microseism in association with human activities, and may potentially contribute to the understanding of spatiotemporal changes of lake hydrodynamics. The details of this study can be found in Gu and Shen (2009).
Seismic Anisotropy and Plate Dynamics
Despite significant progress in understanding the lithospheric mantle and the tectonic history of Alberta (see pioneering work by the Lithoprobe project and references therein), the details of the mantle fabric and flow beneath southern and central Alberta remain enigmatic. Data coverage has been, until recently, a key factor since receivers either had limited depth sensitivity or followed linear geometries. By forming a relatively uniform grid, the CRANE array adds a new dimension, literally and figuratively, to the investigation of crustal/mantle structures beneath our region.
The need to improve our knowledge of the regional history and dynamics has rarely been questioned. Tectonically, Alberta represents an important part of western Canada, a diverse geological framework containing Archaen craton(s), Proterozoc orogens and accretionary margins (known as ‘terranes’). Its extensive strain history, which includes episodes of faulting, magmatism, accretion and subduction (Hoffman, 1988), could have been permanently imprinted onto mantle rocks. A direct manifestation of past strain and the ongoing deformation is seismic anisotropy, owing mainly to the preferential alignment of olivine’s crystollographic fast axis with the direction of maximum shear or least compression at mantle depths.
To study anisotropy we select multiple Mw>6.5 earthquakes recorded at every CRANE station and examine shear wave splitting (Bowman and Ando, 1987; Silver and Chan, 1991; Menke and Levin, 2003), a phenomenon often interpreted as the consequence of anisotropy similar to optical birefringence of minerals under polarized light (see review by Long and Silver, 2009). The splitting parameters, which consist of delay time (between the fast and slow shear waves) and azimuth, directly reflect the strength and direction of anisotropy. In our analysis each measurement is made based on the cross-convolution Method (Menke and Levin, 2003) and then independently confirmed by the rotation correction method (Bowman and Ando, 1987), minimum energy method (Silver and Chan, 1991) and eigenvalue method (Silver and Chan, 1991). The delay-time and azimuth uncertainties are determined by a bootstrapping re-sampling algorithm (Efron and Tibshirani, 1991) based on randomly selected subsets of earthquakes.
A preliminary analysis of the CRANE array data shows clear evidence of shear wave splitting. The shear wave particle motion after correcting for anisotropy is approximately linear, as expected for an isotropic Earth (Figure 7); only a single anisotropic layer is needed to linearize the particle motions. Splitting parameters vary in a systematic manner across the CRANE array (Figure 8). The majority of the measurements along the Rocky Mountain foothills (e.g., CLA, LYA, NOR, BRU) exhibit a northwest-southeast orientation and consistently large (~1.5-1.9 sec) time delays, whereas substantially lower splitting time delays are observed beneath easterncentral Alberta. The SKS fast orientations in the latter region are approximately tangential to a north-south trending ellipse centered at 200-300 km northeast of Edmonton.
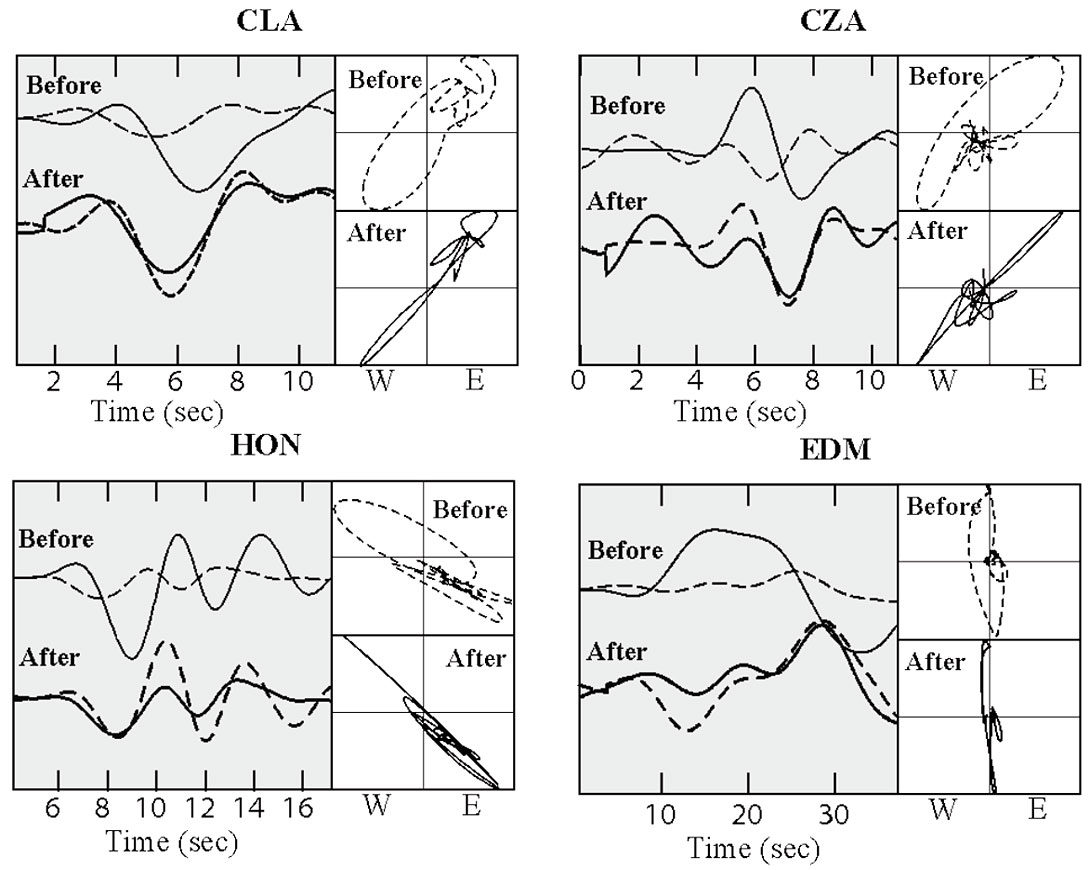
The SKS splitting parameters (i.e., direction, amplitude) in the vicinity of the Canadian Rockies suggest strong ‘order’ in mantle mineralogy (Long and Silver, 2009). The northeast-southwest trending fast direction is consistent with earlier reported values based on Lithoprobe data (Shragge et al., 2002). It is also in general agreement with the direction of maximum horizontal stress – a proxy for the ‘fossil’ strain field within the lithosphere in response to past episodes of northwest-southeast convergence, the most recent being the subduction of the Farallon and Kula plates (e.g., Helmstaedt and Schulze, 1989; Ross et al., 2000). Coincidentally, the fast SKS splitting direction is parallel to the absolute plate motion of the North American continent. The combination of pre-existing fabric within the mantle lithosphere and the present-day, plate-motion induced shear within the asthenosphere offers an attractive explanation for the large SKS delay times and 3-6% azimuthal anisotropy in this region. Without further data constraint it is nearly impossible to reliably resolve the number of anisotropic layers (e.g., Shragge et al., 2002; Marone and Romanowicz, 2007).
The origin of the anomalous shear-wave splitting patterns beneath eastern-central Alberta remains enigmatic. It could potentially be linked to the adjacent Buffalo Head Terrane, an area that has attracted national attention in recent years due to the discovery of precious minerals and potentially economic diamonds. The vicinity of the anisotropic anomaly also exhibits enhanced heat-flow (Blackwell and Richards, 2004; see Figure 8) and below-average seismic velocity (Van der Lee and Frederiksen, 2004; see Figure 8) and bouguer gravity values. The presence of a divot (Fouch et al., 2000) or an abandoned plume conduit (Bank et al., 1998) on the continental root offers a viable explanation. According to this hypothesis, a geometrical imperfection associated with past plate interactions could entrap hot asthenospheric material and, at the same time, disrupt the mantle flow around it. Alternatively, the anomalous shear-wave splitting observations in eastern-central Alberta could be the mantle expression of a hidden tectonic boundary between stable continents (east/northeast) and accreted terranes (west). In this scenario, streamlined mantle flow around the edges of moving continental `keels’ (e.g., Gaherty and Jordan, 1995; Ben Ismail and Mainprice, 1998; Bokelmann and Silver, 2002) could induce strong north-south oriented horizontal strain. Both radial and azimuthal anisotropy would be expected, as well as major complexities in shear-wave splitting directions.
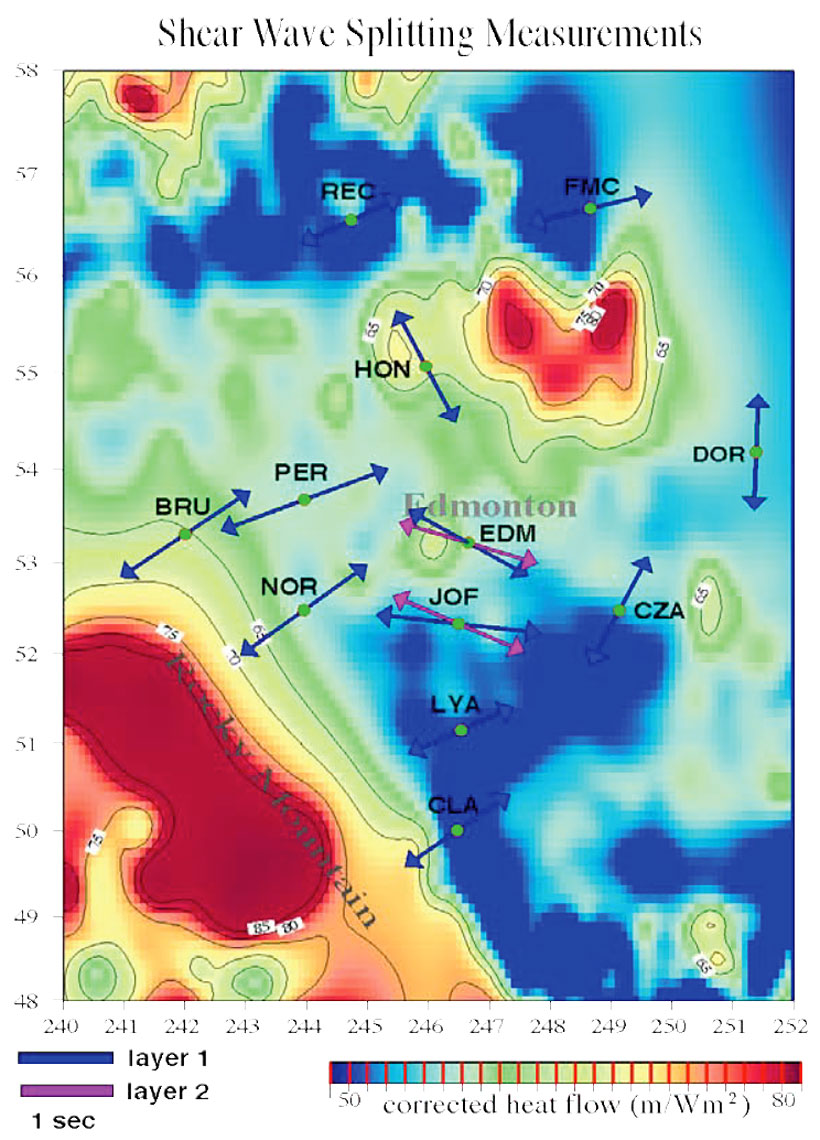
The aforementioned shear wave splitting results show only a glimpse of the laboratory component of our infrastructure project. Further details of this analysis could be found in Gu et al. (in preparation, 2009). Regardless of the interpretations, the data from CRANE present a window of opportunity to examine the present state and geometry of the continental lithosphere. And these results may ultimately contribute to the discussion of the mechanism(s) by which lithosphere evolves from thin convergent margins to thick, depleted continental roots. We are optimistic that, by integrating with the various ongoing research efforts, the nature of the anomaly presented here will become clearer in the near future.
Concluding Remarks
The establishment of the CRANE array opens a new chapter in regional seismic monitoring and crust/mantle imaging in Alberta. The array deployment schedule and the geometry complement the USArray project, the leading international initiative with dense seismic and magnetotelluric arrays presently deployed south of our province. The quality data records from CRANE have shown significant promise in source and structure determinations, as evidenced by the results presented in this article. It is our hope that this project will foster further in-province and cross-border, multi-disciplinary collaborations. Additional information pertaining to the array project can be found in recent Edmonton Journal article “Alberta’s seismic pulse gets a reading” (June 10, 2009) and Rocky Mountain House Mountaineer article “Nordegg Earthquake research aids Alberta scientists.” (June 23, 2009).
Acknowledgements
We sincerely thank Andy Langlois, Len Tober, Christian Escalante and Yuanjie Huang for their contributions during the development of the seismic array. We are indebted to all the hosts of our seismic stations for their generous support of this project. Their passion for basic scientific research in Alberta is exemplary. We also thank Mauricio Sacchi, Doug Schmitt, Martyn Unsworth and Claire Curry for constructive scientific discussions, as well as the technical staff at CNSN for their assistance on data retrieval. This research is supported by CFI, Alberta Ingenuity, National Science and Engineering Council (NSERC) and the UofA.
Join the Conversation
Interested in starting, or contributing to a conversation about an article or issue of the RECORDER? Join our CSEG LinkedIn Group.
Share This Article