This Science Break is a 2-for-1 article special! Our regular columnist Oliver Kuhn tackles the timely topic of mRNA Vaccines, and RECORDER Format Editor Elizabeth Atkinson steps out from behind the scenes with her section on Molecular Testing. Combined they cover two important aspects of the fight against the Covid-19 virus. Besides highlighting some fascinating science, they also provide excellent examples of how scientific R&D investment and effort, and understanding genomes, can result in significant advances and improvements in the quality of human life.
mRNA Vaccines – by Oliver Kuhn
As we know, scientific advances usually follow a slow, laborious path, involving many incremental steps forward along with inevitable dead ends, but occasionally punctuated by glorious breakthroughs. The development of mRNA vaccines has been relatively rapid and fuelled by enormous levels of money and research effort. That has resulted in an amazing breakthrough recently with the delivery of tested and ready for distribution mRNA Covid 19 vaccines in less than a year from the start of the outbreak.
It has been obvious for some time, especially within the medical community, that the human species was increasingly vulnerable to mass pandemics. This is a topic which my article on bacteriophages touched on (Kuhn, 2019). Bacteria which are dangerous to humans have become increasingly resistant to antibiotics, and recent viral epidemics, such as SARS and Ebola, suggested that it was just a matter of time before some animal virus mutated and jumped from its traditional animal host species to infect humans, and had just the right combination of transmissibility and mortality rates to pose a serious threat to our species. And then Covid 19 came along.
Vaccines have been an incredibly effective weapon against diseases (Fig. 1), but they have their limitations and drawbacks. For one thing, they take a long time to develop and test. To date, smallpox is the only disease to have been eradicated with vaccines, and it took over 200 years for this to happen. Admittedly, while the first smallpox vaccine was developed in 1796, most of the critical advancements occurred from the 1950’s on, until eradication of smallpox was achieved in 1980. More illustrative of vaccine development time is that prior to the Covid 19 vaccine, the fastest vaccine development was that for mumps, and that took about 4 years. In that time a pandemic such as Covid 19 can run rampant, so clearly, we need to find a way to develop vaccines more quickly.
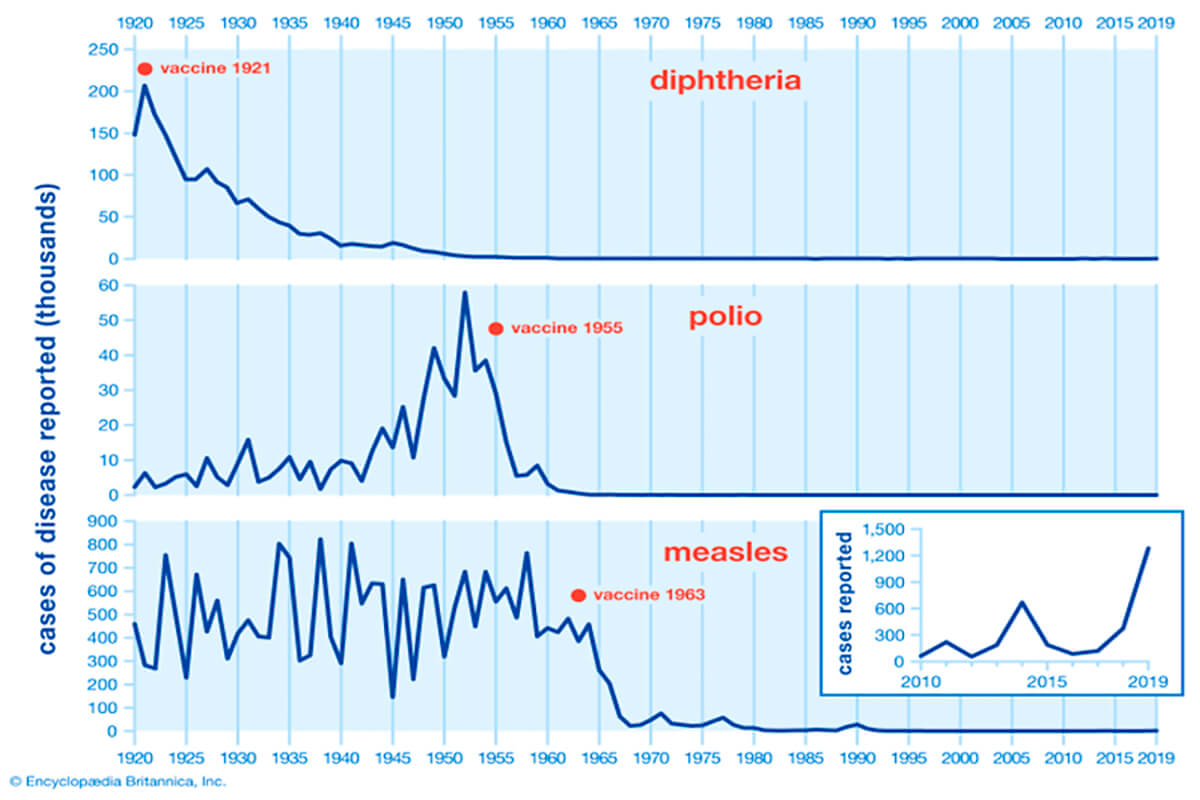
Another weakness of conventional vaccines is that often they are ineffective against pathogens which are able to mutate, which as we’ve seen with Covid 19, viruses are very good at. The large-scale production and distribution of conventional vaccines can also be challenging. Lastly, conventional vaccines are largely ineffective against non-infectious diseases such as cancer. So, the medical research field has been searching for a vaccine solution which addresses some or all these shortcomings.
Messenger RNA (mRNA) technology first appeared around 1990, but due to various significant challenges involved in its use, the search for more effective vaccine methods largely ignored it until about a decade ago. Since then, research progress has accelerated, and when Covid 19 showed up it was perfectly poised for a major R&D effort to push mRNA vaccines across the finish line.
In this article I will first summarize how conventional vaccines work, then cover various aspects of mRNA vaccines.
Conventional Vaccines
All vaccines work on the same principle – they stimulate the body’s natural immune response so that if/when it is infected by the actual disease the vaccine is targeting, the body is ready to deploy its defense mechanisms and fight off the pathogen. A number of vaccine approaches are possible, and they have become increasingly more sophisticated over time. There is some evidence that smallpox inoculation was first used in China around 1,000 years ago, then India around 1550, Liberia, Africa in 1600, and the Ottoman Empire, even Wales, later in the 1600’s. These efforts to combat smallpox were more like folk remedies than anything resembling modern medicine, and involved taking pus from smallpox scabs and introducing it into the body by pinpricks, or blowing it into the person’s nose. This was done both as a treatment of already sick people and as a preventative measure with healthy people yet to be infected. Obviously, this approach risked infecting the person with the disease in addition to inducing the desired immune response.
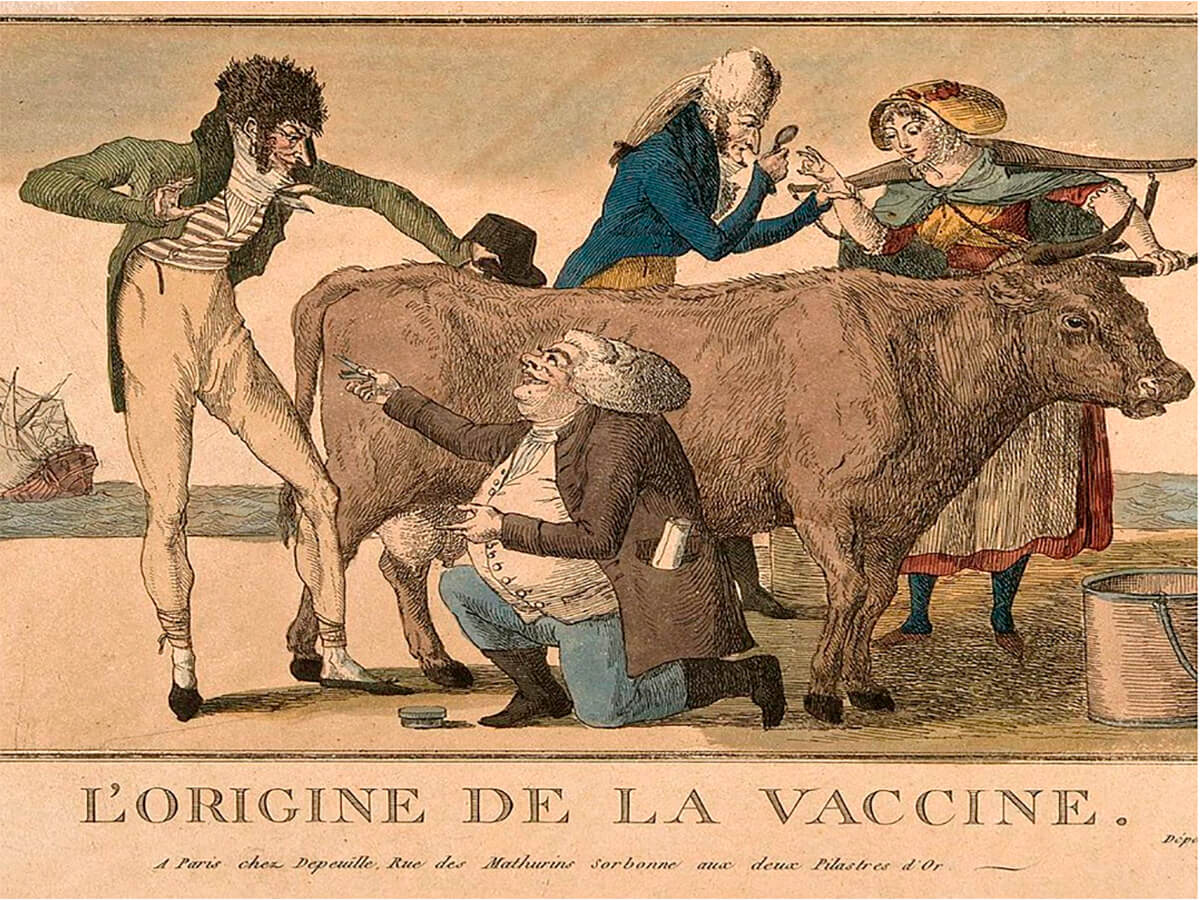
Edward Jenner (1749–1823) (Fig. 2) is generally considered to have delivered the first safe smallpox vaccine, in 1796. What he did was insert cowpox pustules into people; cowpox is not a serious danger to humans but is close enough to smallpox to trigger an effective immune response against it. He did not just randomly place his test subjects at possible risk. He learned or observed that the milkmaids in his native Gloucester who were infected with cowpox, obvious from the pustules on their forearms, were immune to the smallpox epidemics which ravaged England at this time. Inspired by this he collected pus from some of these milkmaids and smeared it into cuts he made on the arms of his test subjects, initially young boys. This proved to be successful, and the rest as they say, is history. An interesting note is that the word “vaccine” is derived from the Latin adjective “vaccinus”, meaning cow like or from the cow.
This method of inoculating against smallpox was quite successful and became widely used. In 1885 Louis Pasteur developed a rabies vaccine, and that really kick-started the science of bacteriology, which then delivered a large variety of vaccines and antitoxins to fight against the many diseases which afflicted humans in the 19th century, including anthrax, cholera, diphtheria, plague, tetanus, tuberculosis, and typhoid. Since then, most advancements in vaccine science have been refinements in the production, storage, delivery and effectiveness of all these vaccines, along with of course, the introduction of a few new vaccines, such as for polio, mumps, measles and rubella (and many more for animals, which has greatly assisted the meat industry, not to mention helped fight disease among pets; there are even vaccine-like methods used to fight pathogens which afflict food plants).
How do vaccines work? All vaccines contain some material that is designed to trigger the desired immune response without risking the health of the recipient. Vaccines can be categorised based on their active ingredient:
- Whole pathogen vaccines
This is the most primitive and risky form of vaccine. Since the actual disease is being introduced to the subject’s body, the risk is they will get the full-blown disease. The trick is to introduce just a small amount of the pathogen, so the immune response is triggered and defeats the disease, and is then ready to face a “real” infection. This approach is not used in modern medicine. - Heterotypic vaccines
Jenner’s use of cowpox pustules as a vaccine against smallpox in humans is a classic example of this type of vaccine. Material from an animal disease is used, similar enough to the human disease to trigger an effective immune response, but different enough to be safe for humans. The BCG vaccine used against tuberculosis is a currently used heterotypic vaccine; it is derived from Mycobacterium bovis, the bacteria which causes bovine tuberculosis. - Live attenuated vaccines
This represents the logical progression from whole pathogen vaccines. Here the vaccine contains whole versions of the targeted bacteria or virus, attenuated or somehow altered in such a way that a healthy person will not get ill from it, while still triggering the immune response. Of the conventional vaccines, live vaccines typically create the strongest and longest lasting immune response, so they can be very effective. However, if given to a person weakened by some pre-existing condition, the virus or bacteria in the vaccine can multiply too much or too rapidly and cause sickness in the person. So there is some risk associated with this type of vaccine. Some types of shingles and chickenpox vaccines are examples of this approach. - Inactivated vaccines
In these vaccines the bacteria or virus used as the active ingredient has been rendered dead or inactive, so it is incapable of replicating, thus it is impossible for the recipient to be infected with the actual disease. The downside is that the immune response can be weaker and shorter lasting than those triggered by live vaccines. Some polio, hepatitis, and rabies vaccines are examples of these ones. - Subunit vaccines
These vaccines do not contain any whole bacteria or viruses, rather their active ingredients are specific antigens, often referred to as “flags”, which are taken from the surface of the target pathogen, usually polysaccharides (sugars) or proteins. These vaccines also tend to create a weaker, shorter immune response, so they are often helped along by booster shots, and the use of adjuvants. Adjuvants help boost the immune response, and are often the cause of side effects such as swelling and soreness in the vicinity of the injection. - Recombinant vaccines
These vaccines are at the high-tech end of the spectrum. Pieces of DNA are taken from the targetted disease, viral or bacterial, and a yeast or bacteria is used to replicate the DNA fragments. The product is purified into a concentrated form which can then be readily deployed via a vaccine. These vaccines address some of the drawbacks of the other approaches, achieving strong and lasting immune responses without some of the risks and side effects seen with live, attenuated and subunit vaccines. - Toxoid vaccines
With some bacterial diseases it is not the actual bacteria that pose the health risk, it is toxins they release. The body’s immune system recognizes these toxins in much the same way as it does a pathogen, so vaccines with safe, attenuated versions of the toxins, known as toxoids, are used. Thus, an immune response is achieved safely without actually using any bacterial material. Some common toxoid vaccines include those for diphtheria, tetanus, and whooping cough. - Conjugate vaccines
It was found that some vaccines using polysaccharides as their active ingredient did not create a sufficient immune response in children and babies. Researchers found that conjugating (in medicine, a term for joining) the polysaccharide with a toxoid, usually a tetanus or diphtheria toxoid, was a great way to increase a vaccine’s efficacy in this younger demographic. An example of such a vaccine is MenACWY, which conjugates the tetanus or diphtheria toxoids with four types of bacteria that cause meningococcal disease. - Virus like particle (VLP) vaccines
These are naturally occurring molecules that closely resemble viruses, hence their name, and the ones selected for use in vaccines trigger the desired immune response. Because they are not actually viruses, they are totally safe. Recently researchers have been able to synthesize them in ways that they are even more effective, primarily by containing several antigens at once, and / or acting as adjuvants. - Outer membrane vesicle (OMV) vaccines
OMV’s are the basic building blocks for the outer membranes of some bacteria. They are not capable of replication or any other bacterial function – they are simply components yet to be assembled – but they can contain the antigens needed to trigger the desired immune response. This means they can be harvested from bacterial cultures, and then used as the active ingredient in vaccines. This is a recently developed technology, and the only such vaccine I could find in general use is one used against meningococcal B. - Viral vectored vaccines
This method uses harmless viruses to enter the body. These vectored viruses have been modified so that their DNA contains the instructions to build the desired antigens, along with all the other steps associated with replication. Once the harmless virus is inside the recipient’s cells, it replicates as viruses do, and creates the antigens which trigger the immune response. CRISPR technology is used to do the genetic modification of the virus – see my article on CRISPR (Kuhn, CRISPR Genome Editing, 2016). The AstraZeneca and Johnson & Johnson Covid-19 vaccines currently being deployed are examples of viral vectored vaccines. Both use an adenovirus considered harmless as the vector. - DNA vaccines
Viral vectored and nucleic acid (DNA & RNA) vaccines represent a paradigm shift in vaccine technology. Instead of inserting some kind of antigen or antigen-like material into the recipient’s body, these methods deliver instructions to the recipient’s cells to create the antigens. The first such nucleic acid vaccines to be researched were those based on DNA.
To my knowledge there are no commercially available DNA vaccines yet, but they are getting close. The basic idea is that DNA snippets related to the creation of the desired antigens or flags are inserted into the recipient’s body, the body’s cells absorb these DNA strands, and the antigens are created via normal cellular mechanisms; these antigens then trigger the immune response. CRISPR is used to insert the desired DNA segments. Something called electroporation or electropermeabilization, essentially exposure to low level electrical waves, is used to increase the permeability of the body’s cell membranes, allowing the vaccine DNA to enter the body’s cells. Once inside the cells, the DNA converts to messenger RNA (mRNA), which then issues the instructions to the cells to create the desired antigens.
These antigens are typically protein spikes that the pathogen has on its surface. In a live virus, the spikes are used to bind to and enter the cells of the pathogen’s host, and once inside the cells the virus’s DNA does its thing and hijacks the cells’ genetic processes to replicate itself (the virus) rather than whatever the cell would normally do. When a DNA vaccine causes these protein spikes to be created, they are displayed on the surface of the host’s cells, and so appear just like a pathogen to the body’s immune system, but the spikes are harmless, just a trick to trigger the body’s immune response. - mRNA vaccines
And that brings us to mRNA vaccines… These are very similar to the DNA vaccine approach but bypass the DNA-to-mRNA conversion step, thus they achieve their results more directly. The reason that they have only just now been developed is that RNA is much less stable than DNA, and there were several very tricky problems to overcome before in vivo delivery (i.e., into a living person’s body) was possible. The mRNA Covid 19 vaccines now being delivered are carried within tiny fatty lipid membranes. This membrane protects the unstable mRNA, and once inside the body, fuses with the body’s cellular membrane, allowing the mRNA to enter the cell. Here the inherent instability of the RNA becomes a benefit, because after it issues its instructions to the cell to build antigens, natural cellular mechanisms break down the RNA and its constituents are absorbed and processed by the body in a totally safe manner, same as the way all the other genetic material is continuously being repaired and replaced.
mRNA represents game changing steps forward in several ways. Because the mRNA in the vaccine is incapable of combining with the body’s own DNA genetic code, it is totally safe. Because the mRNA is under the total control of its developers, it can be designed very specifically to create the required immune response, even tailored to different types of patients. This also allows it to be modified fairly quickly to handle new pathogen variants, and to contain instructions for several antigens representing different types of pathogens in one vaccine. Importantly, it can be created in large quantities at a relatively low cost. One drawback is that the vaccines need to be stored at very low temperatures, creating logistical challenges, but these can and have been overcome.
Messenger RNA (mRNA)
What exactly is mRNA, and what are the steps it is involved in?
We all know more or less what DNA is. It’s a double stranded helical molecule that contains all the instructions a life form needs to create itself, a cellular blueprint of sorts. mRNA plays a critical role in carrying out those instructions to create the proteins the lifeform needs for, well pretty much everything – cells, enzymes, matrix (collagen, elastin, fibronectin, etc.), blood and all the rest. Everything in the body is made up of molecules constructed from proteins, and the proteins are all built using the instructions carried by the mRNA.
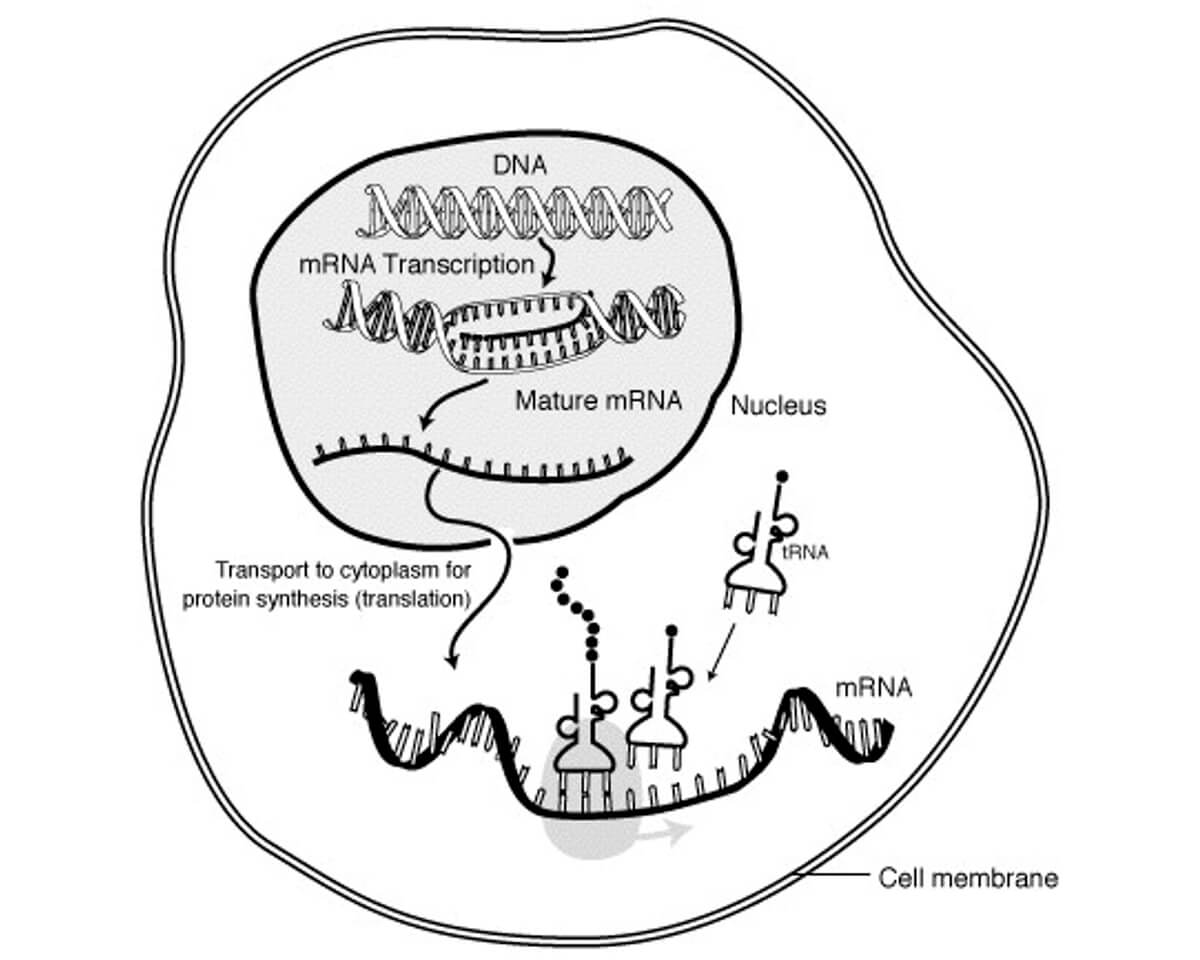
The mRNA role follows a 4-step process, see Figure 3:
- Transcription
Starting with the DNA, the segment with the instructions to build a specific protein is isolated and duplicated as a single strand of RNA. This work is done by RNA polymerase, an enzyme. In eukaryotes (organisms whose cells contain nuclei) such as humans this includes an intermediary step, where the RNA is temporarily double stranded, the second strand being called the transfer RNA, or tRNA. That double stranded pre-mRNA is processed to form mature mRNA, which is single stranded. The tRNA is not discarded; tRNA plays a role later in the process. - Transportation
The mRNA then moves from the nucleus to the cytoplasm area of the cell, which contains ribosomes (something like a catalyst containing an enzyme). - Translation
The ribosomes then translate or “read” the DNA instructions carried by the mRNA, and using constituents found floating around within the cytoplasm, build the desired protein chain (aka polypeptide). In Figure 3 the polypeptide being built is represented by the chain of little black circles coming out of the top of the tRNA in the middle. - The cell then expresses these polypeptides, which go out into the body to perform whatever role they are designed to do. The mRNA and tRNA is broken down by the cell and its components are then ready to be used again.
To expand a bit on the translation part, without getting too deep into the weeds, refer to Figure 3 again. The lower part of the figure, where the three tRNAs are landing on the mRNA like little lunar landing pods, is expanded on in Figure 4.
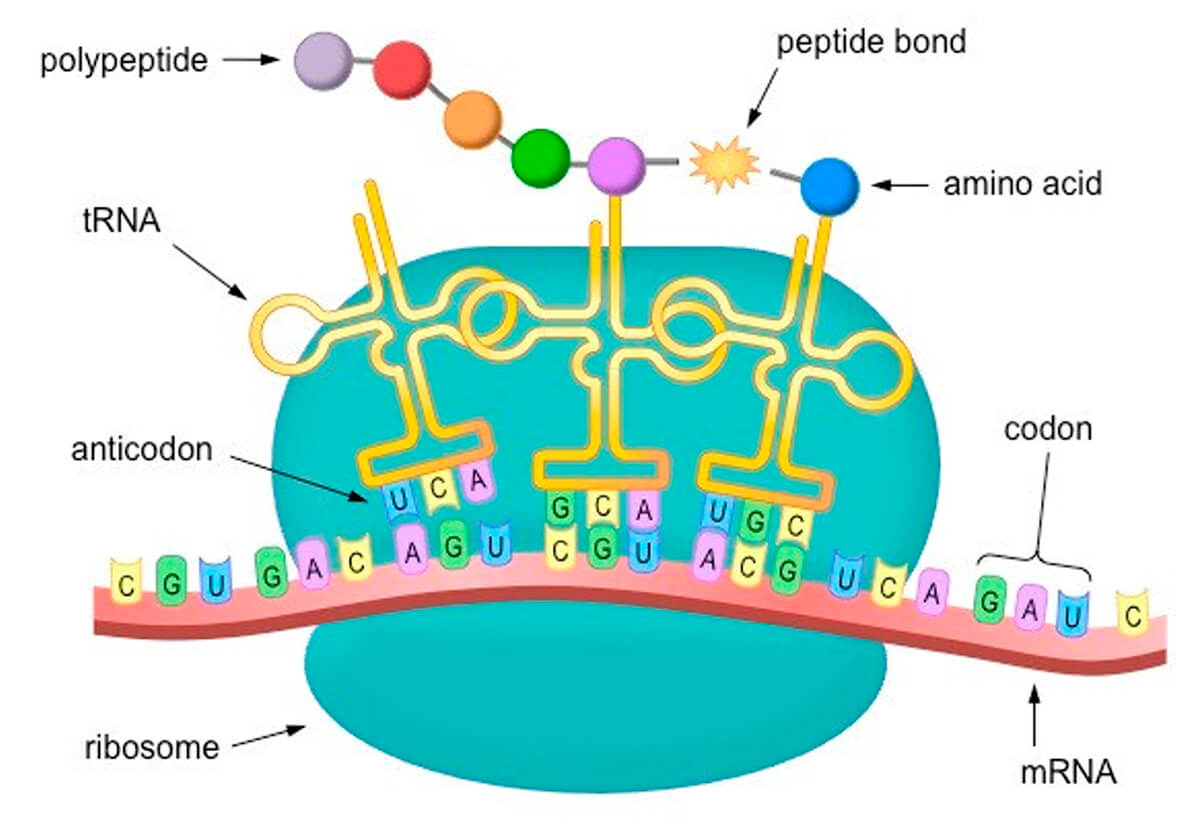
The bases that make up the RNA sequence are four nitrogenous compounds, adenine, cytosine, uracil, and guanine, represented by A, C, U and G. Note these are the same as in DNA, except that DNA uses thymine (T) instead of uracil (U). These are the basic building blocks that make up the nucleic acids found in RNA and DNA. The RNA compounds are sequenced in triplets called codons. The tRNAs carry corresponding anticodons that will bind to a specific mRNA codon, and at their other end they carry an amino acid. An AUG codon in the mRNA is always the starting point, and from there on, in sequence, each codon is paired with its corresponding tRNA anti-codon, which then adds its amino acid to the growing peptide chain, and thus the protein is assembled, all according to instructions.
mRNA as Vaccine
At this point in the article, most of the heavy lifting has been done. It can be readily seen that by using appropriately designed and produced mRNA, an mRNA vaccine will cause our bodies to create the desired antigens, which will then trigger the desired immune response, leaving our bodies well prepared to detect and defeat the disease as soon as it enters our bodies. But how are these designer mRNAs created?
In 2012 Thorsten Stafforst at the University of Tübingen discovered that using enzymes, RNA sequences could be edited. Unfortunately, this was at the time CRISPR technology, which involves editing of DNA, was exploding onto the scene. Stafforst’s discovery generated very little interest, and researchers focused on CRISPR methods for several years. Concerns about DNA editing have emerged since then. They can sometimes cause dangerous immune reactions, and if carelessly used, can permanently alter a genome, causing unwanted mutations. mRNA on the other hand, because it is a temporary, unstable messenger and unable to alter the genome itself, is inherently much safer. So, research focus has shifted to include mRNA methods, and skipping all the many incremental advances which have occurred in the last 10 years or so, brings us to today, where companies have been able to produce effective mRNA Covid 19 vaccines in less than a year, an astounding achievement.
A key advancement was the discovery of a class of enzymes called adenosine deaminases acting on RNA (ADARs). It is these which are used to edit RNA to form the mRNA desired for a vaccine. In the case of Covid 19 vaccines, the mRNA being produced contains the instructions to build the spike protein found on the surface of the Covid 19 virus, technically the SARS CoV 2 virus (Fig. 5), a harmless spike, but enough to signal our bodies to create the antibodies needed to fight the virus.
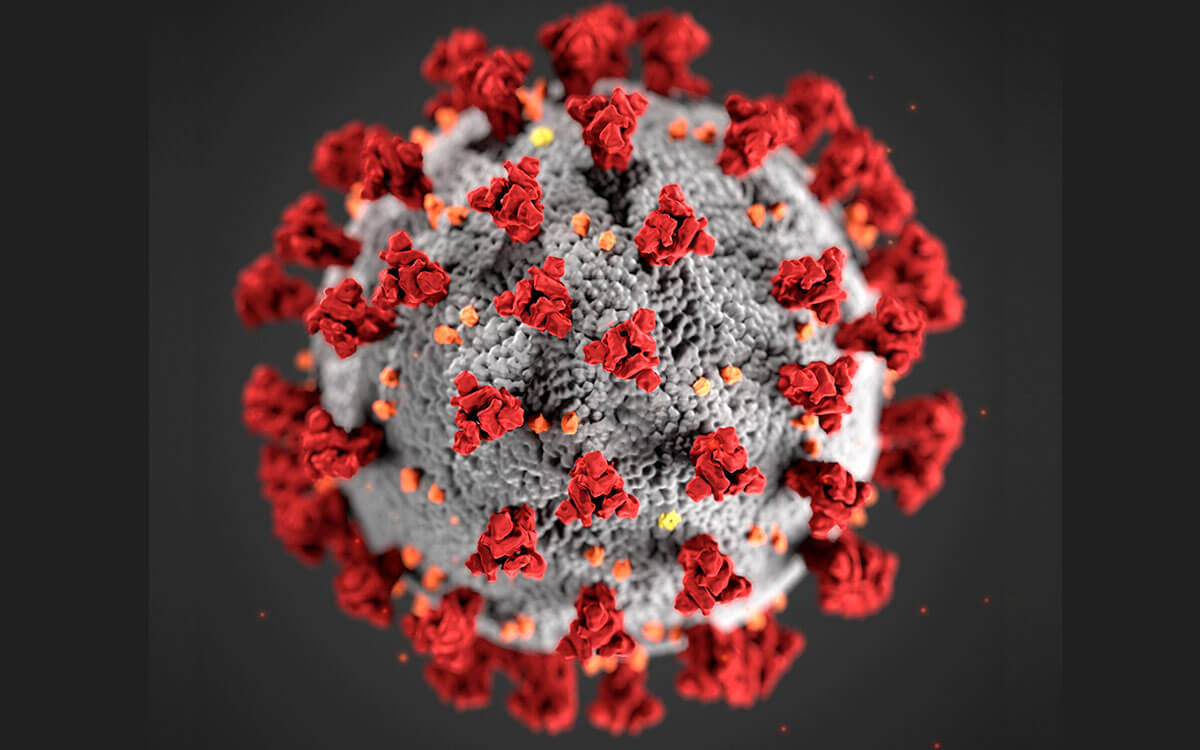
Current mRNA Covid 19 Vaccines
At time of writing, there were two commercially available mRNA vaccines to fight Covid 19, those from Pfizer/BioNTech and Moderna. Mimicking the actual virus, the vaccine is encased in a lipid nanoparticle shell, which allows the vaccine to avoid detection and destruction by the body’s immune system. Because these vaccines were introduced rapidly without a long period of testing, there are unknowns, foremost among them questions about the longevity of the induced immunity. For this reason, both manufacturers are recommending two shots, in an effort to improve the durability of immunity.
Questions also remain about the possibility of autoimmune reactions, which could represent a serious risk to individuals with a pre-existing tendency towards such reactions.
While it is true that the just rolled out Covid 19 mRNA vaccines are the first commercial mRNA vaccines, mRNA vaccines for rabies (CureVac in 2017), influenza (Moderna in 2019), cytomegalovirus (Moderna, 2020), and Zika (Moderna, 2020) have been tested on humans before, with modestly positive results. While the immune responses in these early tests were low, the immune responses to early tests of the Pfizer/BioNTech and Moderna Covid 19 vaccines are high, with percentage resistance to the disease up in the mid-90’s, which is really excellent. Figure 6 shows percentage of infections within a group who received the Pfizer vaccine in two doses (red) and a group who received a placebo instead (blue). It is interesting to note the period of about a week which the body needs to build up immunity; once past that point, the two groups’ infection rates rapidly diverge, a testament to an amazing immunological breakthrough.
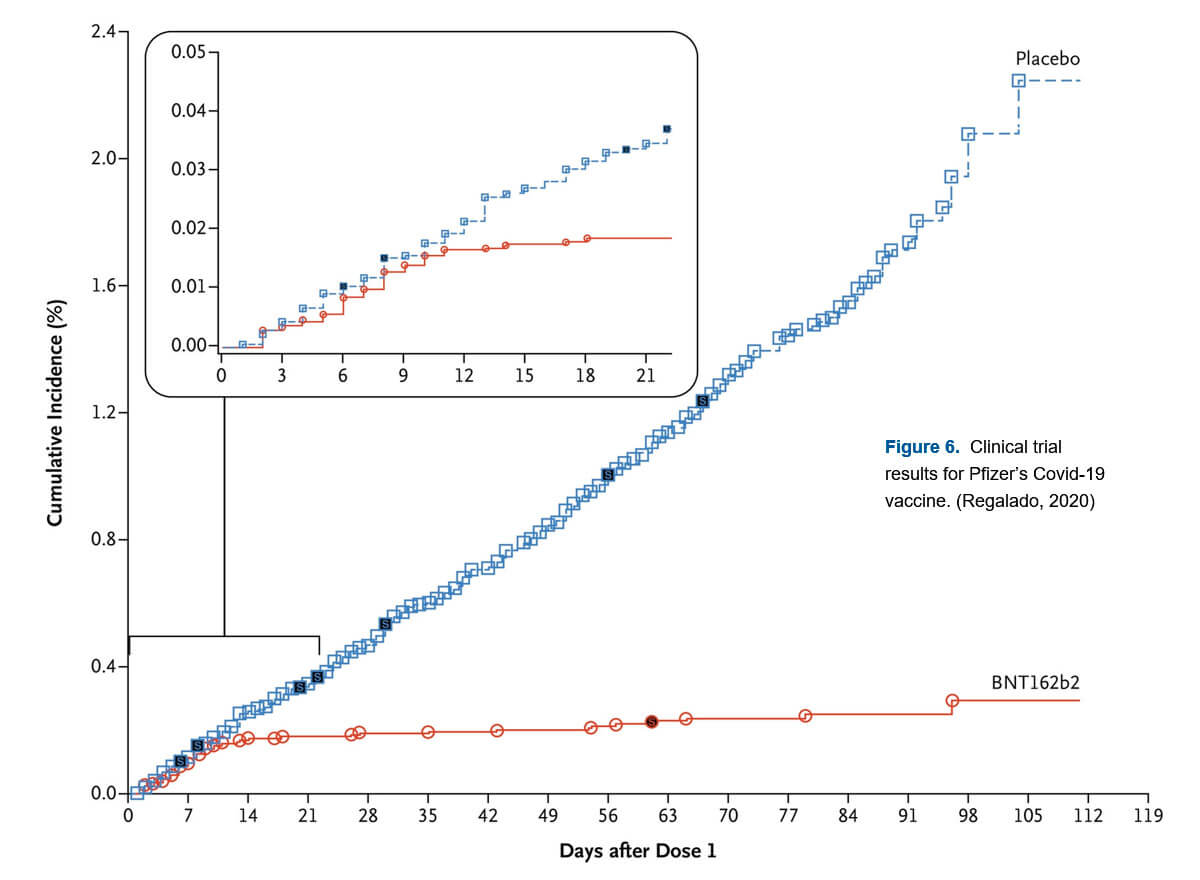
Molecular Testing – by Elizabeth Atkinson
Another modern development that has grown from the understanding of the genome is “molecular testing” for disease. For tracking Covid 19, the most well known and wide spread of these molecular tests is the “PCR” type (“Polymer chain reaction”). Molecular tests use a process similar to what happens when our cells divide, to duplicate DNA, and then detect certain strands of DNA.
When our cells divide, enzymes split the DNA strands and keep them apart, and set a starting “primer”. The enzyme “DNA polymerase” then fills in from the primer with new DNA building blocks (“bases” – A, C, G, and T). Each base in the old DNA strand has a complimentary (not identical) base that attaches to it – A with T, C with G, and when all bases have a new compliment attached, the DNA is fully duplicated (Yourgenome, 2016).
Molecular tests are made to detect low concentrations of RNA or DNA, so they are designed to copy what is there (and not copy what is not there) to “amplify” the amount of genome present. This amplification and the fact that the tests seek specific genome sequences, make molecular tests very accurate. They are both highly sensitive (low false negatives) and highly specific (low false positives). In contrast, antigen tests look for viral proteins and detect them using antibodies on test strips. Antigen tests tend to be highly specific (low false positives), but are typically less sensitive (significant potential for false negatives) (FDA, 2020, 2021).
All Covid 19 tests start with taking a sample by swabbing the nose (best) or back of the throat. For molecular tests, the swab is put in a buffer that breaks open the virus shell and releases the viral RNA into solution. The solution is put into a test-tube with a “soup” of material containing enzymes, individual DNA building blocks (bases), “primers”, and “probes”. The first enzyme, “reverse transcriptase”, takes the viral RNA and makes a DNA equivalent of it.
Molecular tests are designed to look for parts of the genome that are unique to the virus being sought – like fingerprints. The “soup” has strands of bases that fit either side of each “fingerprint”, called “primers”. For a PCR test, the solution is heated to break apart the strands of the DNA (“denaturation”, Fig. 7, Wikipedia, 2021). Once the strands are split, as the solution cools, the primers attach to the strands and fill part of the DNA (annealing). The primers prevent the original strands from reattaching and provide a point from which to build. DNA polymerase then starts at the primers and fills in the blank parts with the loose bases (extension), completing the copy and doubling the amount of DNA. For PCR, this heating and cooling process is repeated 20 to 40 times – called “thermal cycling” – to rapidly amplify the concentration of the DNA in the sample. This takes time and expensive equipment.
Also in that “soup” in the test-tube are DNA strands called “probes”, that are a compliment of the “fingerprint” genome sections, and have the potential to bind to them. These probes also have attached to them a “quencher” and a “fluorophore” (a substance that will glow, if not quenched). IF and ONLY IF the “fingerprint” DNA sequence is present, the probe will attach itself to that “fingerprint” (Tiner, 2020, see illustrations therein). The probe will then be “in the way” of the action of the DNA polymerase as it tries to build the DNA copy; the polymerase will then “eat” through the attached probe, releasing the fluorophore (R.G. Atkinson1 , pers. comm., Jan.19, 2021; Tiner, 2020). Once released, the fluorophore will be separated from its quencher and will fluoresce. Thus, if the virus is present, the sample glows with a certain colour. Tests are designed to seek multiple unique “fingerprint” sections of DNA at once, which helps guard against missing the target if a certain section of DNA were to mutate.
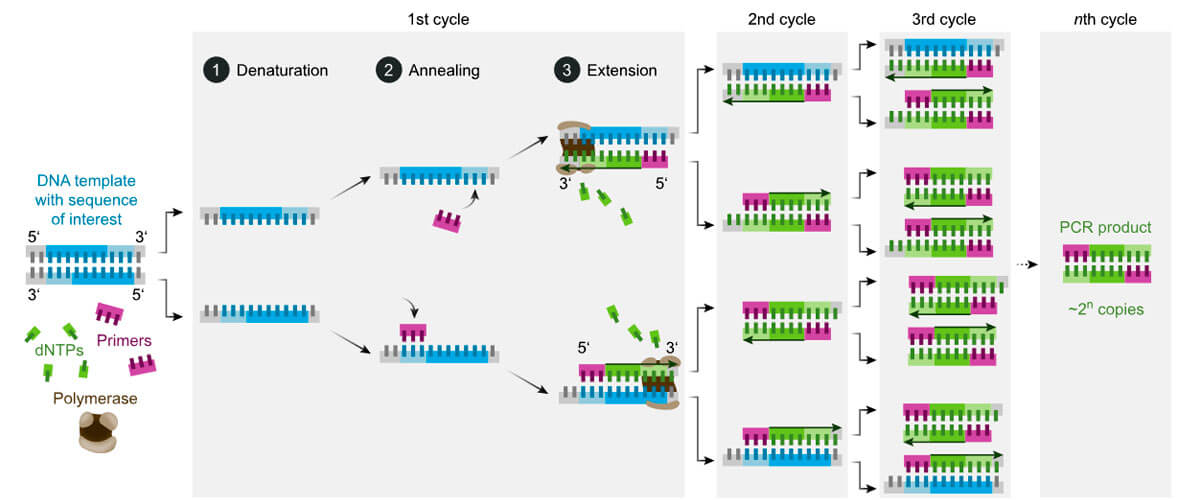
To increase accuracy further, some new tests run a slower control reaction in the same test tube. A sample of a known DNA sequence is included in the “soup”, and probes are included that will detect that control sample and release a fluorophore with a different colour to the target fluorophore. Thus, if conditions are correct and nothing inhibited the DNA amplification process, and the binding and breaking of the probes, then the sample will eventually glow with a colour indicating testing success and a negative result. This control reaction lowers the chance of false negatives, should the test not be run properly.
There are other types of molecular tests in advanced development. Some of these tests use “LAMP” (Loop Mediated Iosthermal Amplification, Wikipedia, 2021) to break the DNA bonds and amplify the DNA. The big advantage of LAMP is that it does not require thermal cycling, so can be implemented as a rapid test (about half an hour, not to be confused with the less accurate antigen tests), with much less expensive equipment. This potential for rapid, inexpensive, wide spread, highly accurate (same standard as PCR) testing has great potential in the fight against pandemics. Some candidates recently received Emergency Use Authorization in the U.S. (FDA, 2021), or are entering trials shortly (R.G. Atkinson, pers. comm., Jan.19, 2021).
All of these molecular tests can be modified quickly to seek out new variants of Covid 19, the flu, or other diseases. Different fluorophores that glow with different colours can be used for each variant or disease, so one test can seek many different things at once, and the test results can be colour coded (R.G. Atkinson, pers. comm., Jan.19, 2021). Molecular tests will play a large part in the future response to pandemics and our “return to normal”.
1 Robert G. Atkinson, Co-Founder of Anavasi Diagnostics, Woodinville, Washington, USA – and author’s brother.
Share This Column