Being one of the least densely populated countries in the world, Canada’s rigorous climate, the energy intensive nature of the country’s industries, and the large distances between population centers result in a relatively high per capita energy consumption. In 2013, for example, Canadians consumed 7.2 tonnes of oil equivalent per capita, which is about four times of the world average (The World Bank, 2017). This amount of energy consumption accounts for about 21 tonnes of CO2 equivalent per capita of associated GHG emissions per year (Environment and Climate Change Canada, 2017) which shows a decrease by 6% from the 1990 level. Based on the Paris Agreement, in 2015, Canada agreed to reduce its GHG emissions by 20% by 2020 and 30% by 2030.This is an ambitious target that can only be achieved by significant GHG emissions reduction in all sectors including buildings.
According to the Office of Energy Efficiency (OEE) of Natural Resources Canada, in 2007 Canadian households were responsible for 16% of the total national end-use energy consumption and 15% of the total GHG emissions (OEE, 2007) (Figure 1). Consequently, any national policy to reduce energy consumption and the associated GHG emissions must address the residential sector energy consumption to be effective. Based on these statistics, it is not surprising to see that an aggressive regulatory agenda including the adoption of up-to-date energy codes for buildings and the labeling of building energy use (so that residential buyers and renters and commercial lease holders can make more informed decisions with respect to energy) is strongly recommended to the government. These changes, combined with the carbon price, will create an important market for energy efficient technology in the residential sectors (Climate Leadership Report to Minister, 2015).
To reduce energy consumption and GHG emissions in the residential sector, different strategies can be considered such as using renewable energy resources, improving end-use energy efficiency, improving envelope and windows characteristics, and introducing alternative energy conversion technologies, such as cogeneration systems that have higher efficiency and produce lower GHG emissions compared to conventional technologies. Among strategies that can be utilized, integration of solar technologies in buildings provides one of the substantial solutions for energy consumption and GHG emissions reduction in Canada’s residential sector.
Solar Technologies for the Residential Sector
Solar energy is currently used for water and space heating, space cooling and generating power for residential buildings. There are a variety of technologies available for solar thermal and power generation in the residential sector, such as solar collectors, photovoltaic (PV) cells and thermal mass. Window upgrade and PV systems reduce the most GHG compared to other investigated upgrades (Nikoofard, 2012). I will detail PV in this paper.
Photovoltaics
Photovoltaics (PV) is a solar power technology that uses solar cells (solar photovoltaic arrays) to convert energy from the sun into electricity. Since the output of a single cell is not sufficient to satisfy a practical load, cells are grouped together to form "PV modules" that may in turn be arranged in "solar arrays" (also known as "solar panels"). Solar arrays are increasingly incorporated into the roof or walls of buildings as a principal or auxiliary source of electrical power. Although the systems are not cost competitive with conventional energy systems, they are attractive for pollution-free generation of electricity. Due to advances in technology and a consequent decrease in manufacturing costs, as well as generous government subsidies, the photovoltaics market has significantly expanded all over the world, especially in the US, Europe, China and Japan. The global production of photovoltaic cells has annually grown at the rate of 30–45% since 2000, and this growth is expected to continue: the global solar electrical capacity in 2000 was 1 GW and is anticipated to increase to 140 GW by 2030 (Zahedi, 2006).
PV systems can be categorized as off-grid or grid-connected. An off-grid system is suitable for remote residential or commercial buildings far from the existing electricity grid, and requires a battery for keeping a balance between electricity supply and demand. On the other hand, a grid-connected system can draw further electricity if needed from the utility grid, and excess electricity can be delivered to the grid (NRCan, 2002; Zahedi, 2006). Therefore, it does not need a battery unit and thus is less expensive compared to the off-grid system. Although off-grid systems were dominating the photovoltaic market at the beginning of the 1990's, the production of grid-connected systems rapidly grew and exceeded the off-grid in 1998 primarily due to generous feed-in tariffs for PV electricity (Hass, 2003).
PV cells suffer from a drop in efficiency with the rise in temperature due to increased resistance. To solve this problem and to utilize the otherwise wasted thermal energy, PV thermal (PV/T) systems were developed. In these systems, heat is carried away by the thermal part of the system, keeping temperatures lower and consequently lowering resistance. When such systems are integrated into the building façade, they are referred to as BIPV/T (Building Integrated Photovoltaic Thermal systems).
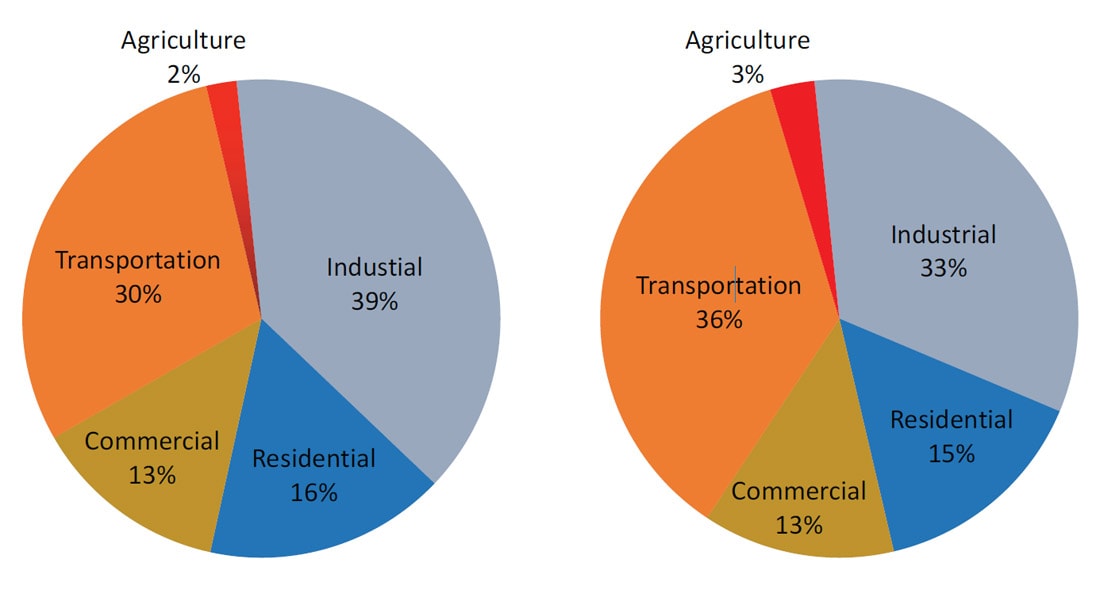
Methodology
The methodology that is used in carrying out this research is depicted in Figure 2 and consists of the following steps:
- Model development/adaption for solar technologies
- Estimation of the annual energy savings and the reduction in GHG emissions for each solar technology upgrade scenario
- Estimation of the tolerable capital cost for each energy efficiency upgrade scenario
- Evaluation of the economic feasibility of upgrade scenarios
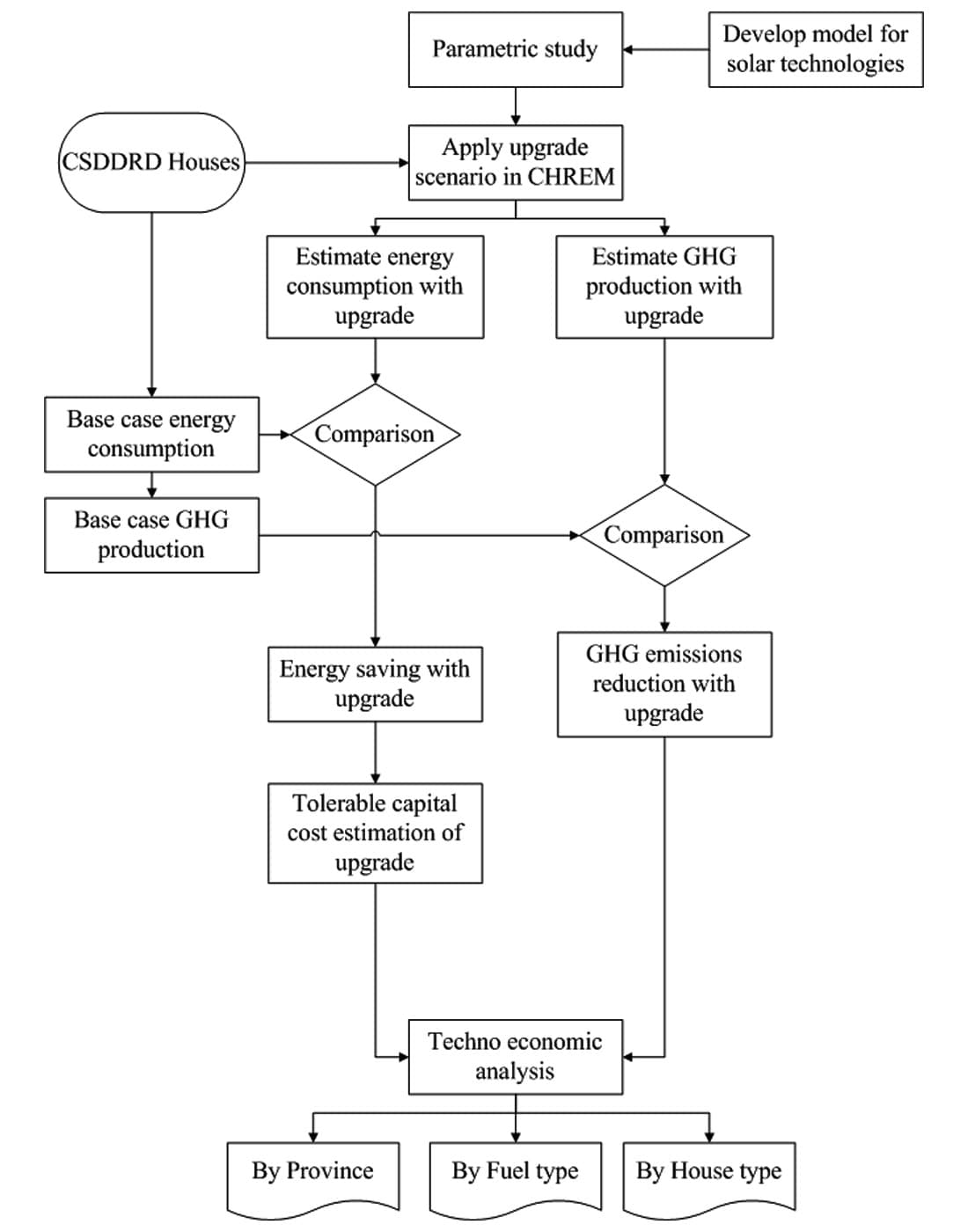
Economic Analysis
In this study an alternative approach to conventional economic feasibility analysis is adopted which involves the calculation of “tolerable capital cost” of the upgrades. “Tolerable capital cost” is the capital cost that one is able to pay based on annual savings, number of years allowed for pay-back, and estimated annual interest and fuel cost escalation rates. Thus, to estimate the tolerable capital cost of each upgrade a reverse payback analysis is conducted (refer to Nikoofard et al. 2015 for details).
Results
The breakdown of energy savings and GHG emission reductions due to upgrading all eligible houses by the addition of PV system are shown in Table 1 for each energy source, house type and province. The results show that the addition of PV systems reduces the energy consumption by 3.4% (representing 35.7 PJ/year) and GHG emissions by 6.4% (representing 3.1 MT of CO2 equivalent). Assuming a CO2 tax of $15/ tonne, this is equal to $46.5 million of levy.
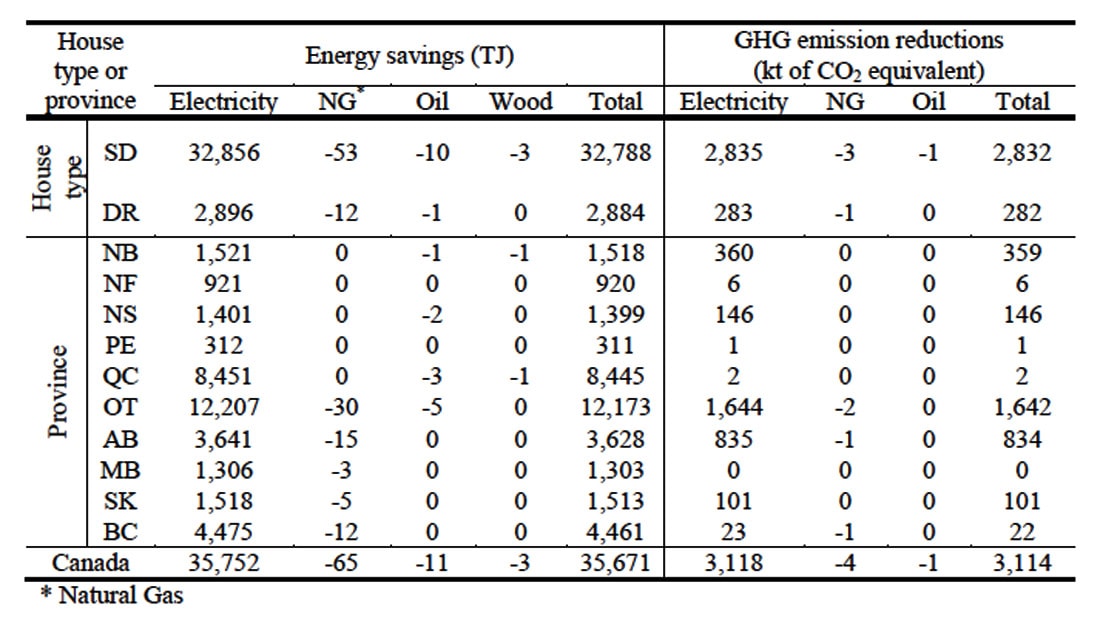
The distribution of energy savings and GHG emission reductions due to the addition of PV systems among the provinces of Canada is shown in Figure 3. This figure shows that the energy savings potential with PV systems in all provinces are similar, while the GHG emission reductions vary significantly. This is due to the differences in the source of electricity. In Quebec for example, there is practically no reduction in GHG emissions by switching to PV systems because of the fact that the electricity used for appliances and lighting is generated by hydro-electric power plants that have no GHG emissions. On the other extreme, the GHG emission reduction is the highest in NB because a large part of the electricity used for appliances and lighting is generated from fossil fuels.
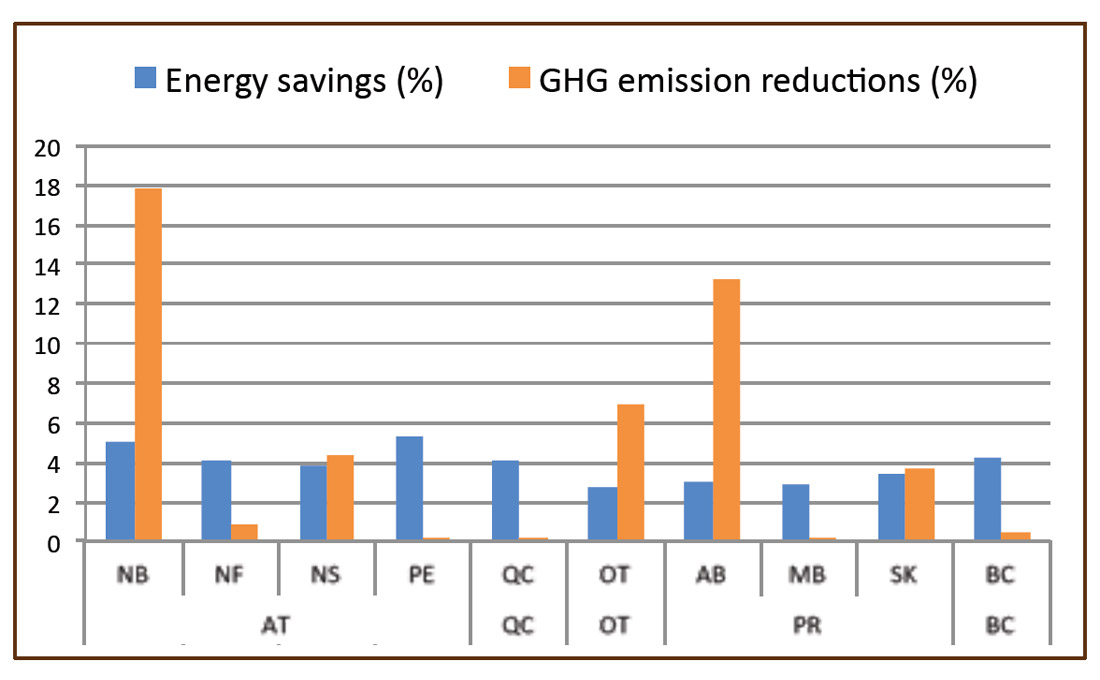
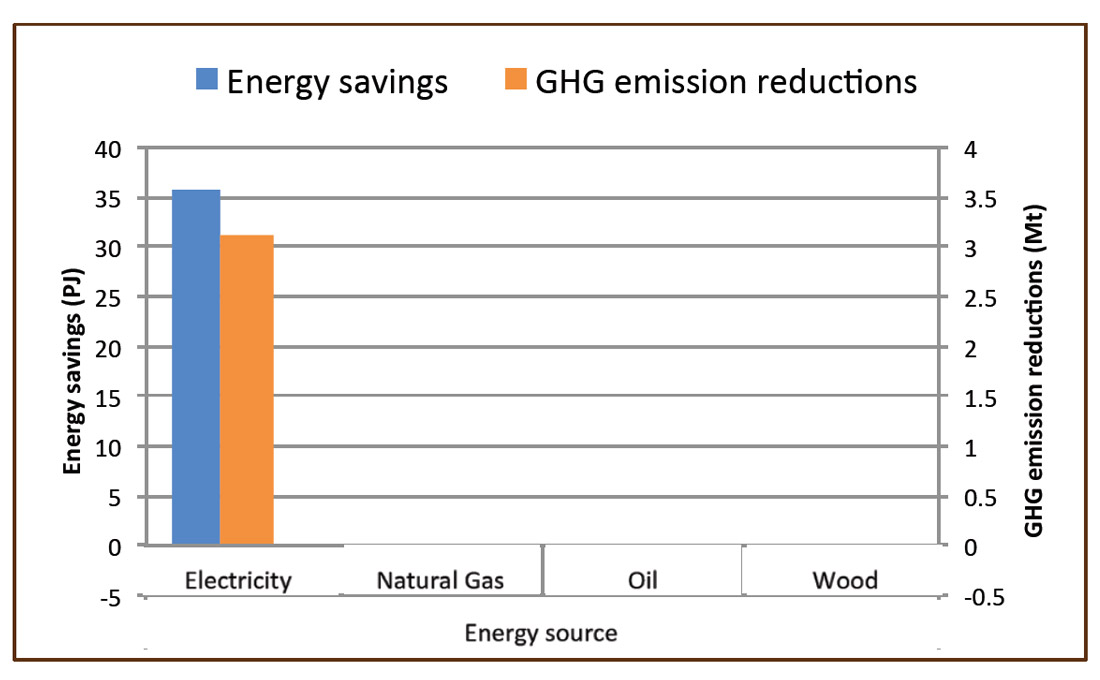
Economic Feasibility of PV Upgrade for the Canadian Housing Stock (CHS)
The estimates of the total tolerable capital costs for three different payback periods, interest rates and fuel cost escalation rates for the PV upgrade are shown in Figure 4.
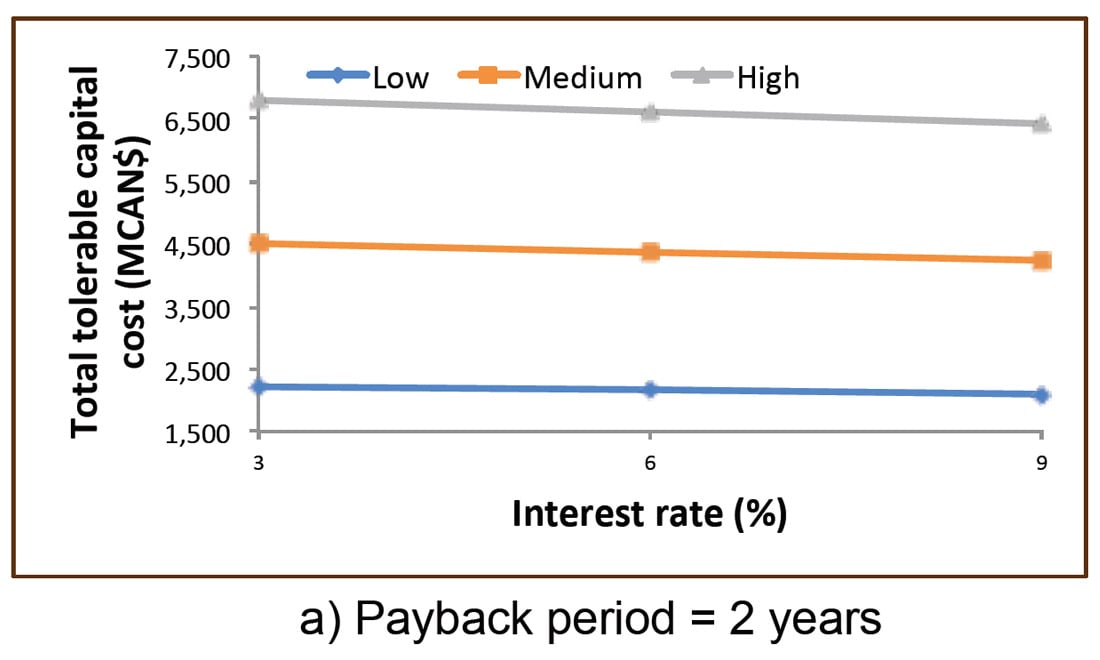
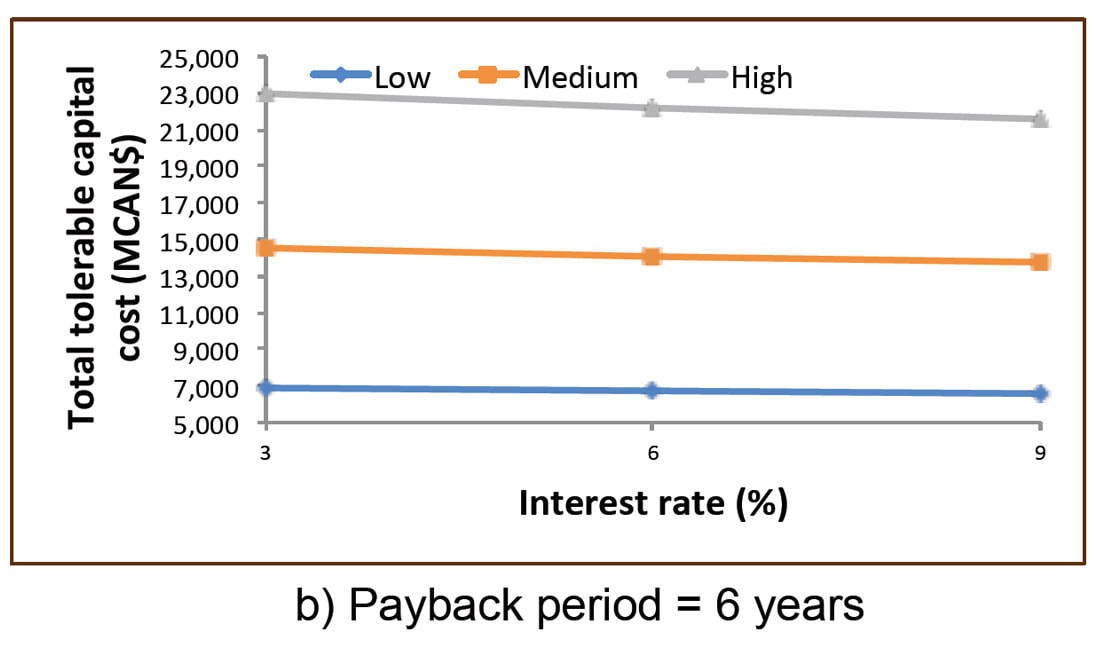
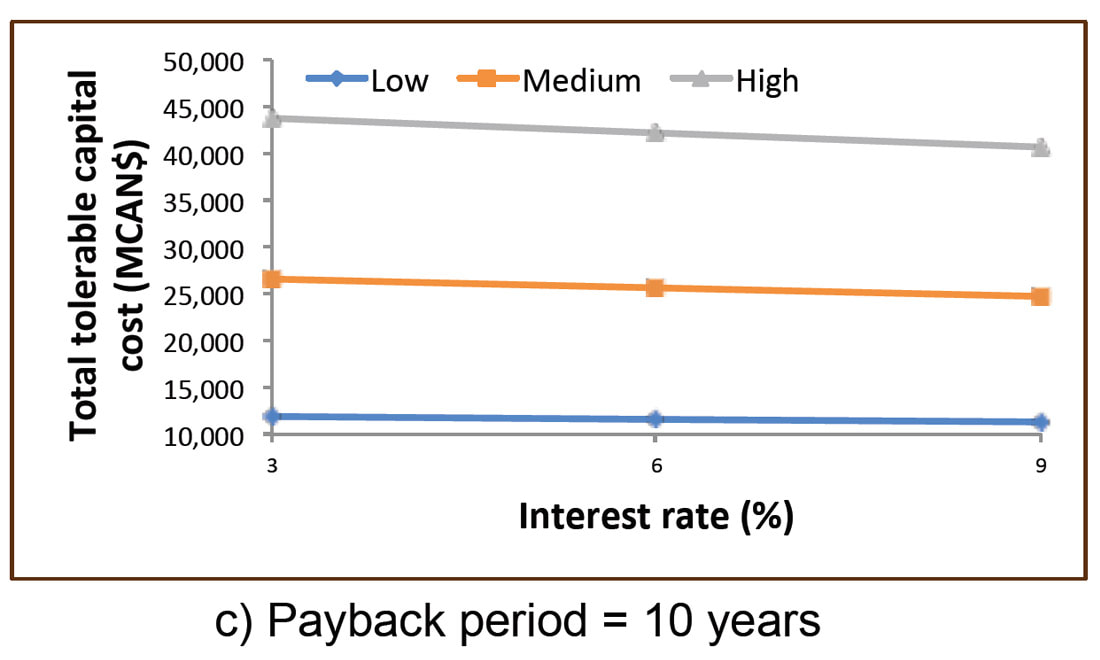
The provincial total tolerable capital cost and achievable savings per house for a 6 year payback period, 6% interest rate and medium fuel cost escalation rate due to PV upgrade is shown in Table 2. The province of NB with an average tolerable capital cost of 3,551 CAN$ per house has the highest upgrading feasibility across Canada. This can be due to a number of reasons such as number of sunshine hours, electricity price, as well as available roof area compared to other provinces.
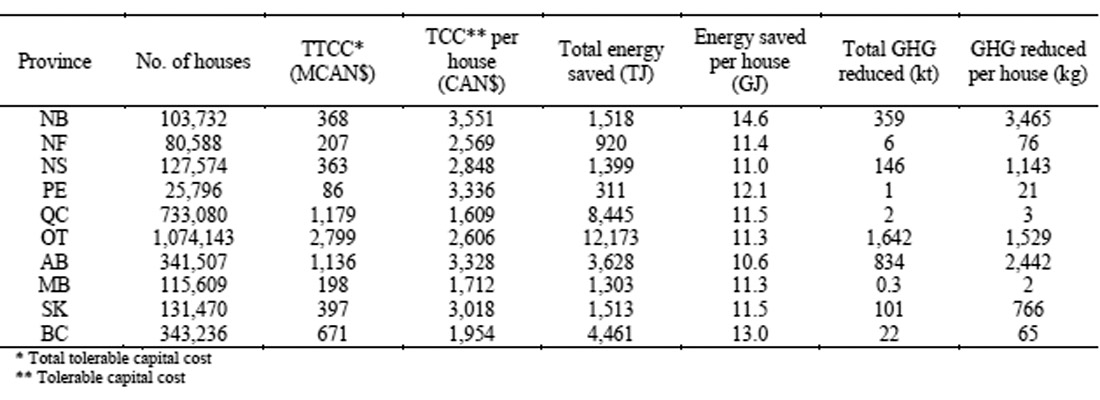
Selected Province: GHG Emission Reduction in Alberta Using Integrated Solar Technologies
The amount of energy savings and the associated GHG emission reductions using integrated solar technologies are detailed for each province in Canada by Nikoofard (2012). Presented in Table 3 are the interesting conclusions for Alberta.
If the primary objective of introducing solar technologies into the housing sector of AB is to reduce the energy consumption and GHGe, the technology to focus on would be PV. This will result in a reduction of energy consumption by 3.672 TJ/yr and will also result in the largest GHG reduction at 834 kt/yr equal to $12.51 million CO2 levy. The tolerable capital cost for this upgrade is $3,328 per household. The second largest potential to save energy is by introducing window upgrade into the province.
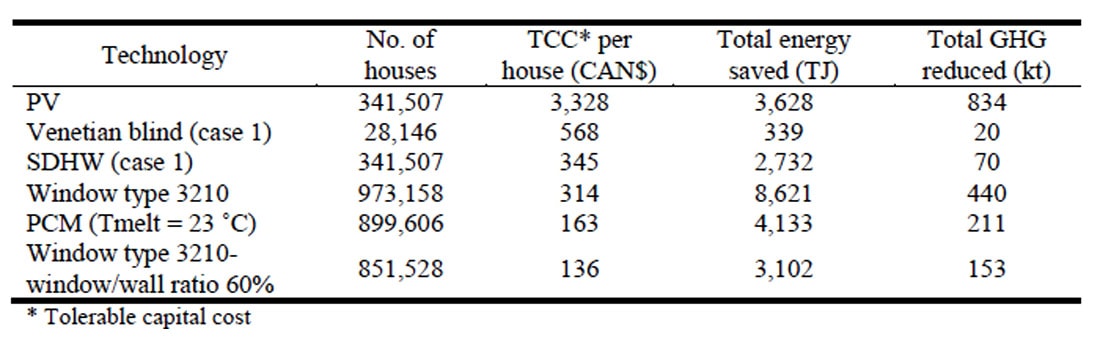
Conclusion
The techno-economic assessment of PV integration to the CHS was presented in this section. Based on the literature review, the PV system was selected for batch simulations. The input data for each component of PV system were selected based on manufacturer specifications. The selected PV system was applied to all eligible houses.
It was estimated that integration of PV systems to the CHS would reduce the energy consumption by 3.4% (representing 35.7 PJ/year) and GHG emissions by 6.4% (representing 3.1 MT of CO2 equivalent). From the economical point of view, upgrading houses to incorporate PV systems in the province of NB is more feasible than other provinces.
It should be noted here that the price of PV modules has decreased by 50% over the past 12 years, and further and substantial reductions are expected (Enerdata, 2012). Thus, the economic feasibility of PV systems is expected to improve over the next decade.
Join the Conversation
Interested in starting, or contributing to a conversation about an article or issue of the RECORDER? Join our CSEG LinkedIn Group.
Share This Article