Introduction
There is a great need for high-quality monitoring data from thermal enhanced oil recovery processes (THEOR – Figure 1) applied to heavy oil reservoirs. We need to understand the physical mechanisms, to improve our attempts at mathematical simulation of these processes, and to provide information for “real-time” project optimization.
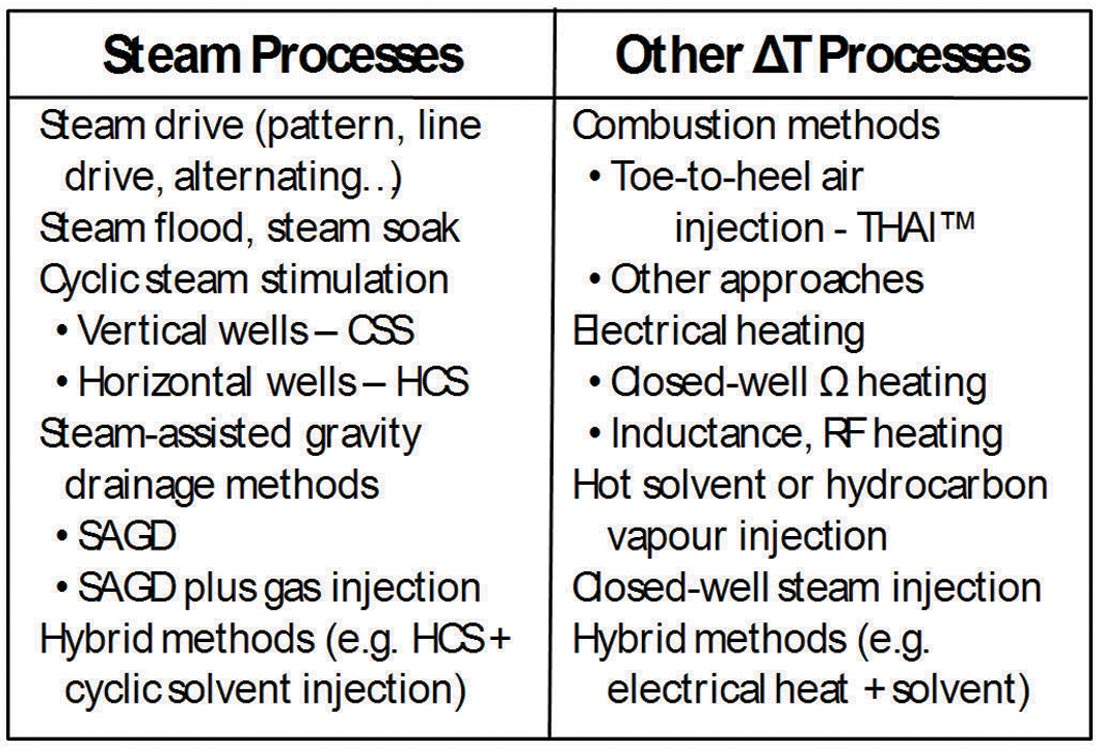
THEOR processes, such as steam injection, generate large changes in amplitude and travel time in the complicated signature of a reservoir’s seismic response. Conventionally, pressure, temperature, and saturation changes induced by thermal recovery are examined to study their complex and often counteracting effects on the seismic attributes of the reservoir and bounding rocks. The effects of geomechanical changes in stress and stiffness are less often considered, though they may dominate seismic property changes. Thus, seismic data interpretation to deconvolve in situ rock properties is non-unique and it also varies with time as these physical parameters change with the maturity of the THEOR process. A fuller understanding of the effects of these changes will lead to better interpretation of changes of seismic attributes, hence better project management.
Considerable enthusiasm exists for application of seismic monitoring techniques ranging from time-lapse VSP, X-hole tomography, and 4-D seismic surveys to microseismic monitoring. Indeed, these methods are becoming integrated as we learn more and more how to use spatiotemporally “random” microseismic events as sources, and as we learn how to introduce various physical constraints into inversion methods.
Nevertheless, there are interpretation difficulties with using only seismic methods and conventional monitoring well data (e.g.: p, T, near-well geophysical response). This brief article is intended to point out some geomechanics issues arising in THEOR monitoring, and to advocate incorporation of more monitoring dimensions.
Thermally-Induced Stress Changes
In steam THEOR processes (Figure 1), temperatures, typically 200°, but as high as 350°C are feasible, giving a ΔT of as high as 325°C. In combustion processes such as toe-to-heel air injection (THAI™), temperatures can reach 500°C to 750°C in the combustion zone, depending on the amount of water vapour present (wet or dry combustion) and the rate and O2- richness of the injected oxidant. The question becomes just how large a stress change could be expected from such a process.
Assuming uniform heating of a flat-lying tabular sandstone reservoir with a coefficient of thermal expansion of 10-5 °C-1 and a confined Young’s modulus of 5 GPa, the increase in horizontal effective stress (Δσ’h) can be estimated to be about 20 MPa for ΔT = 300°C. Because a typical steam process takes place at about 500 m depth, the overburden stress and original hydrostatic pressure are approximately σv =11 MPa and po = 5 MPa respectively, giving an effective overburden stress of Δσ’v = 6 MPa. This may not change much with the heating of a laterally-extensive reservoir since the thermal expansion will be accommodated by surface heave. However, the large increase in σ’h is enough to lead to generalized shearing of the reservoir, particularly since the high injection pre s s u res reduce the confining stress.
Of course, the configuration of heated zones is complex, and induced stress distributions around heated zones show massive increases or decreases in the local σ’3 and σ’1, depending on the geometry and the sharpness of the thermal front. If σ’v were reduced, as would be expected in any high-pressure injection process such as cyclic steam stimulation (CSS) or high-pressure steam-assisted gravity drainage (SAGD), the tendency to yield in shear would be greatly enhanced.
Mathematical modeling shows that yielding can be expected to be a consequence of any process that involves ΔT values greater than perhaps 80-100°C, even in materials such as oil sands that are less stiff than highly competent rock such as a carbonate. In softer rocks, stress changes are less than in stiff rocks, but the stresses needed for yield are far lower because soft rocks – unconsolidated sandstones – are much weaker than stiff rocks such as competent carbonates.
Indeed, there is much independent evidence for shear: amongst other phenomena, early attempts at microseismic monitoring of firefloods in 1981-82 showed clear shear events; casing shear is endemic in CSS projects; laboratory work confirms shearing in oil sands at stress states commensurate with those predicted by simulation; and, ground surface uplifts related to shear dilation are regularly measured.
Shearing, Dilation, and Mechanical Damage of the Rock
Shear and dilation
THEOR induces shearing, which is the failure mode that occurs when the anisotropic stresses in the rock exceed its frictional and cohesional strengths. This results in numerous shear zones within the rock along which sliding occurs. Because of the confinement conditions and the shape of the heated reservoir zones, shear within the reservoir does not occur only on isolated local planes. In unconsolidated formations, this sliding results in the translation, rotation, and displacement of individual sand grains that invariably results in a permanent disturbance of the original granular structure and an increase in bulk volume known as dilation. Shearing is prevalent in shallow unconsolidated reservoirs undergoing THEOR both because of the high differential thermal stresses induced by the process, and by the reduction in effective confining stress with high pressure injection that reduces the frictional strength of the rock. What are the consequences for geophysics?
As the thermal front propagates laterally into the reservoir (Figure 2), σ’h is increased both inside and outside the thermal zone because of laterally-constrained thermal stress within the growing thermal zone. At the same time, σ’v is decreased outside the shoulders of the thermal zone because of thermal jacking: vertical extensional strains due to thermal expansion in the heated zone unload some of the stress within the cold reservoir. For these shallow reservoirs, not only does this make shearing inevitable, it means that any remnant stiffer (unyielded) rock will attract higher stresses by virtue of its higher stiffness, and eventually be forced into a yielding condition.
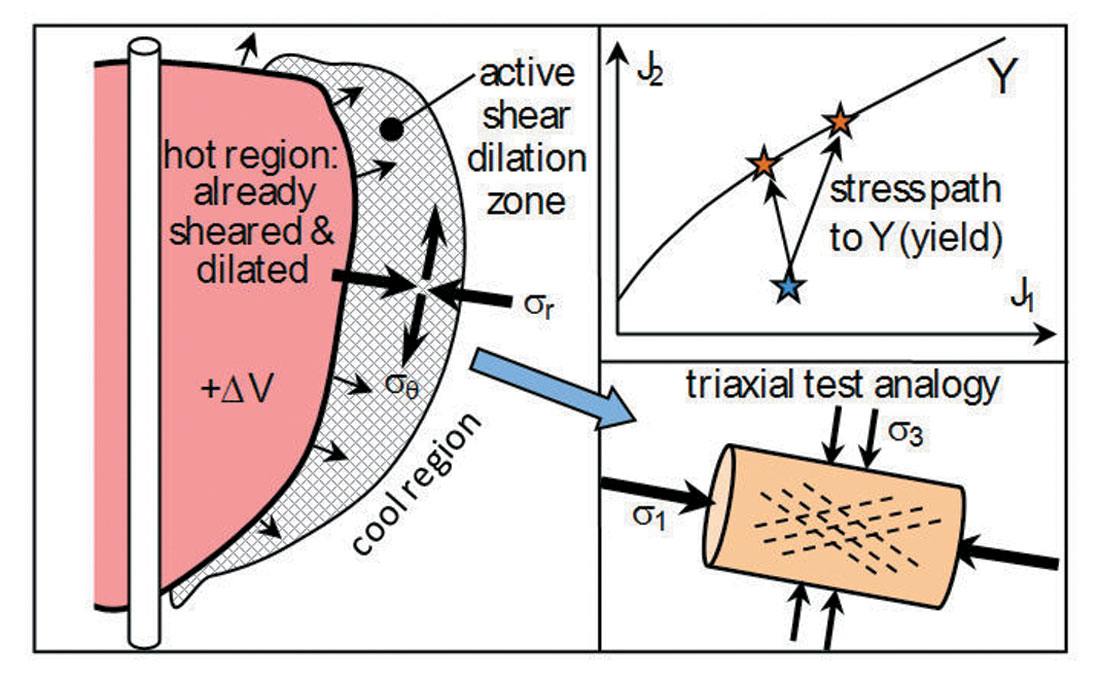
Thus, in advance of the front, almost all the rock shears at low confining stresses. In competent quartzose sands (i.e. most of the world’s oil sands), grain crushing is minimal, and the shear dilation potential is great. For a typical Athabasca oil sand at a confining stress of 1-2 MPa, it is likely that the dilation would approach 5-6%; in other words, the porosity would increase from 30% to about 35% as shear distortion takes place (Figure 3). The consequences on transport properties are huge; not only does the increase in absolute porosity result in an increase of absolute permeability by a factor of 2 to 10, in typical cases with high oil saturation the relative permeability to the mobile water phase is increased by orders of magnitude. Furthermore, shear will break up the thin clay dustings found on bedding planes and between bed sets; macroscopic flow impedance is thus greatly reduced. The success of SAGD and CSS in bituminous sandstone reservoirs can in significant part be attributed to the effects of shear dilation that enhance the transport properties.
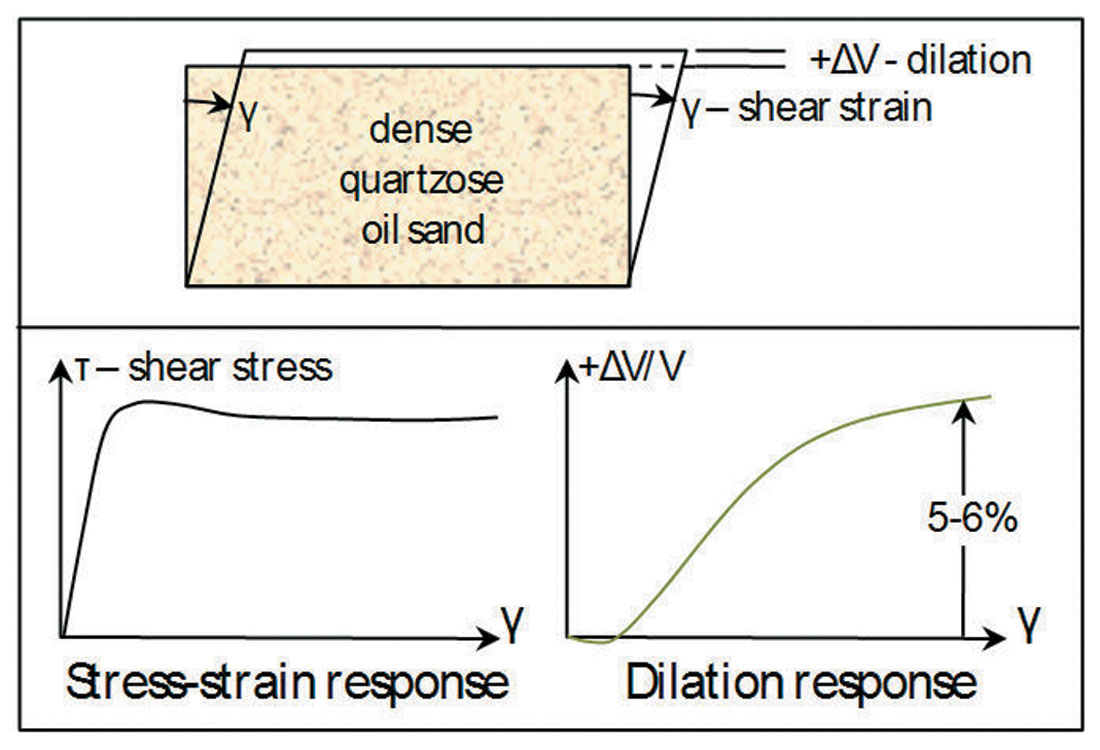
Effective stress
Seismic characteristics will be affected significantly as an indirect result of the increase in relative permeability caused by imbibition due to dilation. The increase in relative permeability ahead of the thermal zone will propagate full injection pressures tens of metres ahead of the thermal zone, into the cold reservoir. These elevated pressures will reduce the effective stress on the rock, within the cold reservoir, thus reducing the seismic transit times.
The changes in pre s s u re and stress cause shear yield, as evidenced by the endemic and continual microseisms associated with THEOR processes, and the stiffness of yielded rock is far lower than for intact rock, drastically increasing the transit times and attenuation, resulting in marked differences in seismic characteristics even in areas beyond the thermal zones. Note that increases in gas saturation during injection are unlikely in these cold areas because the increase in pressure tends to drive the pore liquids towards undersaturated conditions. Solution gas drive is, however, expected to play a dominant role during drawdown.
Shear at the shale caprock
What else happens? The shales overlying a THEOR project are essentially impermeable in comparison to the reservoir. As thermal expansion and dilation take place, a huge shear stress concentration develops between the reservoir and the non-expanding overlying shales, leading to shearing along the interface between the two, and possibly within very weak planes within the shale caprock. This shearing is not favourable, as it is sufficiently large to collapse any penetrating well. Projects such as the Imperial Oil Cold Lake CSS project experience dozens of well shear events yearly; these wells must be repaired or replaced at considerable cost. Methods of reducing the incidence and the impact of shearing have been suggested, such as horizontal wells or changes in the process sequence.
Reservoir Deformations
Deformations in the reservoir cause deformations at the surface. For example, a pure volumetric dilation source gives rise to a monopolar Δz distribution, whereas a shear distortion gives rise to a dipole (Figure 4). For reasonably well-constrained cases – such as a single flat-lying reservoir at constant depth – the surface deformation field can be inverted to give a distribution of volumetric and shear distortions at the depth of the reservoir horizon. In practice, this has been used to help understand production mechanisms and even, in one unpublished case, to predict well shearing arising because of steam injection. Hence, another process-monitoring procedure is available that is complementary to seismic monitoring and also provides a broad examination of the entire project as opposed to localized measurements at observation wells.
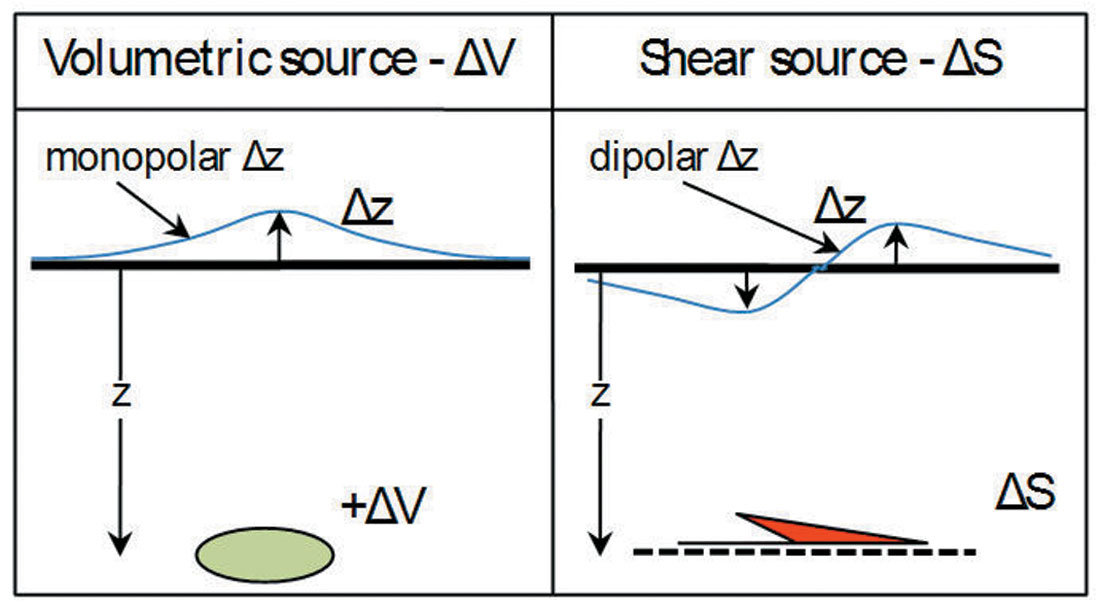
Cyclic injection THEOR projects as well as SAGD projects evidence large vertical surface deformations (Δz) (Figure 5). In THEOR processes taking place at depths of 200 to 500 m, cumulative Δz values as large as 600 mm have been measured after a few years. In fact, for cyclic THEOR processes, Δz is economically important because recompaction is a dominant drive mechanism during late production cycles. This recompaction drive effect increases oil recovery rates, and likely the ultimate recovery factor as well.
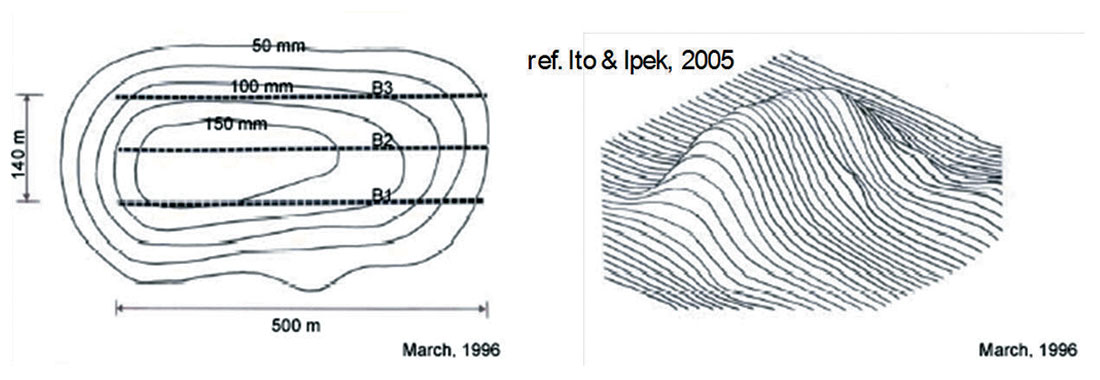
To what processes can a total vertical heave of 600 mm be attributed? Sandstones typically have a coefficient of thermal expansion of about 10-5 °C-1; assuming only thermal expansion, a 200°C average increase in a 30 m reservoir should give Δz ~ 60 mm, one-tenth of the measured amount. All of the rest of the permanent deformation can only be explained by dilation; there is no other viable explanation. Hence, if we can track volume changes in the reservoir by some indirect means such as deformation inversion coupled with seismic data analysis, we can also track important process parameters such as the homogeneity of the process, changes in permeability, and the distribution of swept zones. The implications for learning and process control are clearly quite large and economically interesting.
Impact of Geomechanics on Seismic Monitoring
What happens when a seismic wave passes through a sandstone, and what changes might arise because of changes in the fabric, saturations, or intrinsic conditions? This turns out to be a remarkably complex question for unconsolidated sandstones subjected to THEOR for the following reasons:
- Massive changes in saturation take place, with free gases replacing liquids in the pores.
- Density changes take place because of increasing gas saturations, but also because of dilation
- Stress changes take place because of the thermally-induced volume changes as well as any changes arising because of injection and production.
- Vital but less appreciated, reduction in rock stiffness occurs because of
- the reduction in effective confining stress with increased pressure, and
- the drastic loss of stiffness once the rock experiences yield and dilation.
These changes greatly affect all seismic parameters such as velocity, amplitude, frequency content, and birefringence, thus changing all calculated seismic attributes.
For example, it is common for the shear wave amplitude to disappear in pressurized regions during the injection phase of CSS above fracture pressure because grain-to-grain contacts and effective stresses disappear. In strongly dilated zones, compressional wave amplitudes are reduced, high frequencies are totally filtered out (e.g. if a microseismic event is used as the source), and any initial horizontal shear wave anisotropy is likely eliminated. However, during drawdown periods, effective stresses are re-established, and shear wave transmission becomes possible again, albeit in a rock material that is much softer than it was originally.
Unfortunately, there is no rigorous model that allows the relationships among seismic parameters and various changes to be quantified to a level that will permit deconvolution of the stress field or the dilation field, as examples. Also, there are severe challenges and limitations that arise in attempting to reduce uncertainty through careful laboratory tests. This is because of core disturbance in unconsolidated sands, scale effects , boundary conditions that are different in the field, difficulties in testing at elevated temperatures, and because of issues such as wavelength scale and dispersion.
Suggestions
We believe that careful reservoir and process monitoring pays for itself many times over in terms of enhanced understanding and process control. Also, if it were possible just to optimize injection and production rates, temperatures and times based on independent monitoring data, recovery factors in THEOR processes would undoubtedly rise. The way forward requires several actions:
- Integrate geomechanics simulation explicitly with thermal flow simulation, including the effects of dilation and stress changes.
- Include deformation monitoring (Figure 6) and coupled inversions with seismic monitoring. With time, the data from deformation measurements will help constrain and therefore refine seismic attribute extraction.
- Develop better seismo-thermomechanical models so that it becomes feasible to do forward modeling of seismic parameter changes, which can then be verified in the field, calibrated, and used to give better constraints on seismic monitoring data inversion in THEOR cases.
- Consider incorporating other independent measurements that can have a synergetic benefit to data analyses. Some suggestions are:
- Extension of the precision of deformation inversion by including more precise deformation measurements at the surface and with depth in multi-purpose observation wells.
- Gravity field measurements through use of surface and borehole gravimeters.
- Electrical impedance tomography using a 3-D array of electrodes.
- Better down-hole pressure measurements at high temperatures.
Different disciplines in the geosciences intersect only irregularly. In geomechanics, we too often ignore the value that geophysics and flow simulation can bring. Conversely, we are strong in measurement methods that are not commonly used in petroleum engineering or geophysics, such as deformation measurements, and we understand aspects such as dilation and stress . Disciplinary integration has clear benefits to all parties, and the result will be better interpretation, greater efficiencies, and more value for the investment in monitoring.
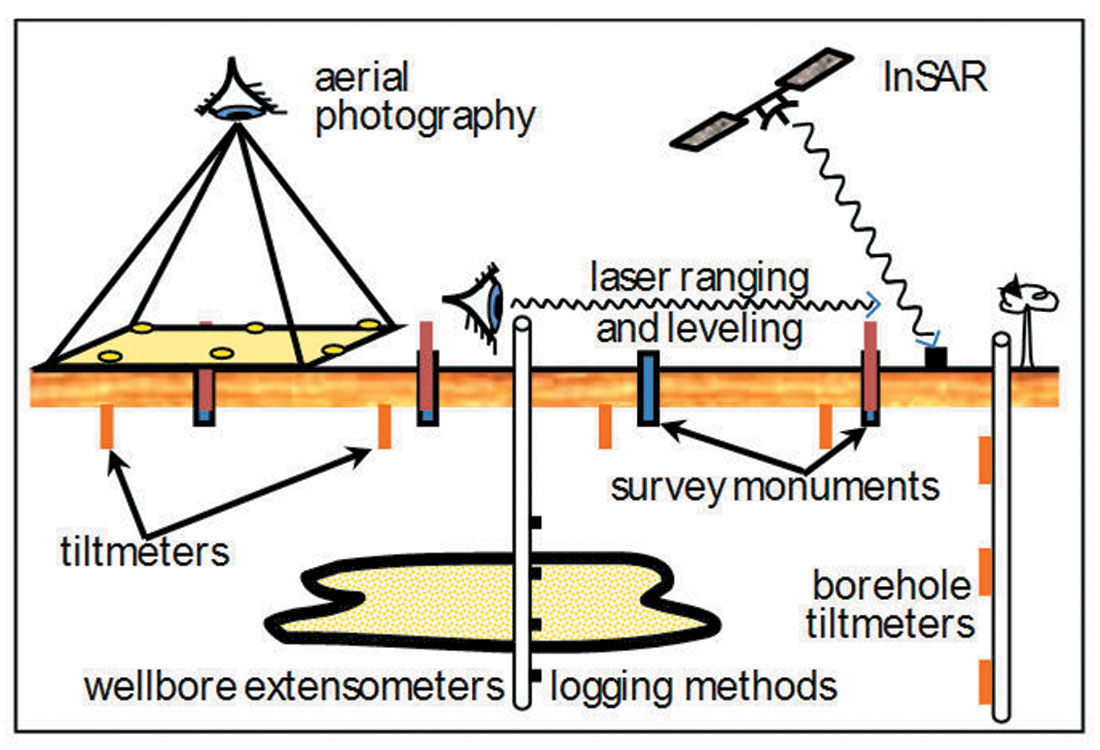
Recommended Reading
The Journal of Canadian Petroleum Technology and the annual Conferences of the Petroleum Society of CIM in Canada are major sources of geomechanics and recovery information, particularly for heavy oil and bitumen recovery.
For geophysics and thermal recovery, see:
Schmidt, D.R., 2004. Oil Sands and Geophysics, CSEG Recorder, Nov., pp.5-11
Schmidt, D.R., 2005. Rock Physics and Time-Lapse Monitoring of Heavy-Oil Reservoirs, paper PS2005-435, SPE/PS-CIM/CHOA 98075, proc. joint CHOA/CIPC/SPE ITOHOS, 1-3 Nov., Calgary, Alberta, 6pp.
Maxwell S.C., Du, J., Shemeta, J.E., Zimmer, U. and Boroumand. N., 2007. Monitoring SAGD Steam Injection Using Microseismicity and Tiltmeters, paper SPE 110634-MS, proc. 2007 SPE ATCE, Anaheim, CA, 11-14 Nov., 7pp.
For some discussion of geomechanics, monitoring, and thermal processes, please see:
Collins, P.M., 2007. Geomechanical Effects on the SAGD Process, SPE Reservoir Evaluation & Engineering, V10N4, August, SPE 97905-PA
Dusseault, M.B., 2007. Monitoring and modeling in coupled geomechanics processes. Proc. CIPC, Calgary, Paper 2007-028, 10 p
Deformation inversion is discussed in:
Dusseault, M.B. and Rothenburg, L., 2002. Deformation analysis for reservoir management. In Oil & Gas Science and Technology – Revue de l’IFP, v 57, No 5, pp 539-554.
Ito, Y. and Ipek, G., 2005, Steam Fingering Phenomenon during SAGD Process, paper PS2005-324 and SPE/PS-CIM/CHOA 97729, proc. joint CHOA/CIPC/SPE ITOHOS, 1-3 Nov., Calgary, Alberta,, 9pp.
Some issues related to geomechanics simulation and geophysics are discussed in:
Dusseault, M.B., Yin, S., Rothenburg, L., and Han, H.X., 2007. Seismic monitoring and geomechanics simulation. Leading Edge, June 2007, 610-620.
Test data on oil sands has been of dubious quality because of massive sample disturbance from gas expansion. For the general science and some test data on seismic velocities in oil sands, works by Amos Nur at Stanford and Carlos Santamarina at Georgia Tech are recommended; please visit their websites for full publications lists. Also, Larry Lines in geophysics at the University of Calgary and Doug Schmidt in geophysics at the University of Alberta are excellent sources of good information.
Join the Conversation
Interested in starting, or contributing to a conversation about an article or issue of the RECORDER? Join our CSEG LinkedIn Group.
Share This Article