In a case study from the Austrian Alps, the capability of Common-Reflection-Surface processing (CRS) for comprehensive fault imaging and analysis is demonstrated. CRS processing is used to generally improve the signal-to-noise ratio and to regularize the dataset in different domains. We show an offset-azimuth regularization to generate well populated gathers in six different azimuth classes. Each azimuth class is separately Kirchhoff-migrated to image faults according to their orientation. For a Reverse Time Migration (RTM) a dataset is shot-receiver regularized. The CRS signal enhancement generally improves the resolution of the subsurface structures, while the regularization minimizes operator noise.
Introduction
Seismic resolution is often limited by a low signal-to-noise ratio, and by numerous data gaps and coverage irregularities. Various processing strategies to reduce these problems are provided by CRS processing based on the Common-Reflection-Surface method. CRS attributes calculated from the seismic wavefield describe the reflection events in detail, and allow to improve the seismic signal over random noise. In the vicinity of existing data, the detailed CRS event description may also be used to reconstruct missing traces. This approach cannot only be applied to fill data gaps, but also to regularize data in different domains.
In a case history from the Alpine Mountains in Austria, we test the advantages of CRS offset-azimuth regularization, and of CRS shot-receiver regularization for different imaging and interpretation aspects. The offset-azimuth regularization is used to generate well populated gathers in different azimuth classes that are migrated separately. The major faults of the subsurface are resolved in all azimuth classes, whereas small, more subtle features are imaged in individual classes with more profound orthogonal illumination.
Offset-azimuth regularization optimizes subsurface coverage which customizes the data for Kirchhoff type migrations, but does not preserve a surface acquisition geometry. Shot-receiver regularization, on the contrary, optimizes the surface coverage by a regular acquisition geometry. This provides a regular distribution of input data for migration in the shot domain, but does not fully normalize the subsurface fold. However, Reverse Time Migration (RTM) as a sophisticated two-way wave equation in shot domain, should benefit from a regular surface coverage.
Method
A sophisticated strategy of time domain stacking of seismic data beyond NMO stacking was first presented by Gelchinsky (1988), taking into account a complex lateral inhomogeneity of the subsurface. Based on these ideas, the common reflection surface (CRS) stack was then designed by Hubral et al. 1999, Mann et al. 1999, and Jäger et al. 2001. CRS stacking is a robust and powerful methodology for seismic data enhancement that has been successfully adapted to exploration seismic during recent years, e.g. Eisenberg-Klein et al. 2008, Trappe et al. 2011, and Pruessmann et al. 2012.
The principle of CRS stacking is summarized in Fig. 1. The seismic data is parameterized according to dipping and curved reflector elements in the subsurface that generate the acquired reflected wavefield. For each event, CRS wavefield attributes related to a point source and to an exploding reflector segment are derived directly from the seismic data. These CRS attributes describe the travel time of a reflection event across several CMP locations. Due to this extended validity, these CRS traveltime surfaces hardly coincide with random noise. As a result, the seismic image is cleaned from random noise, showing an improved resolution and event continuity.
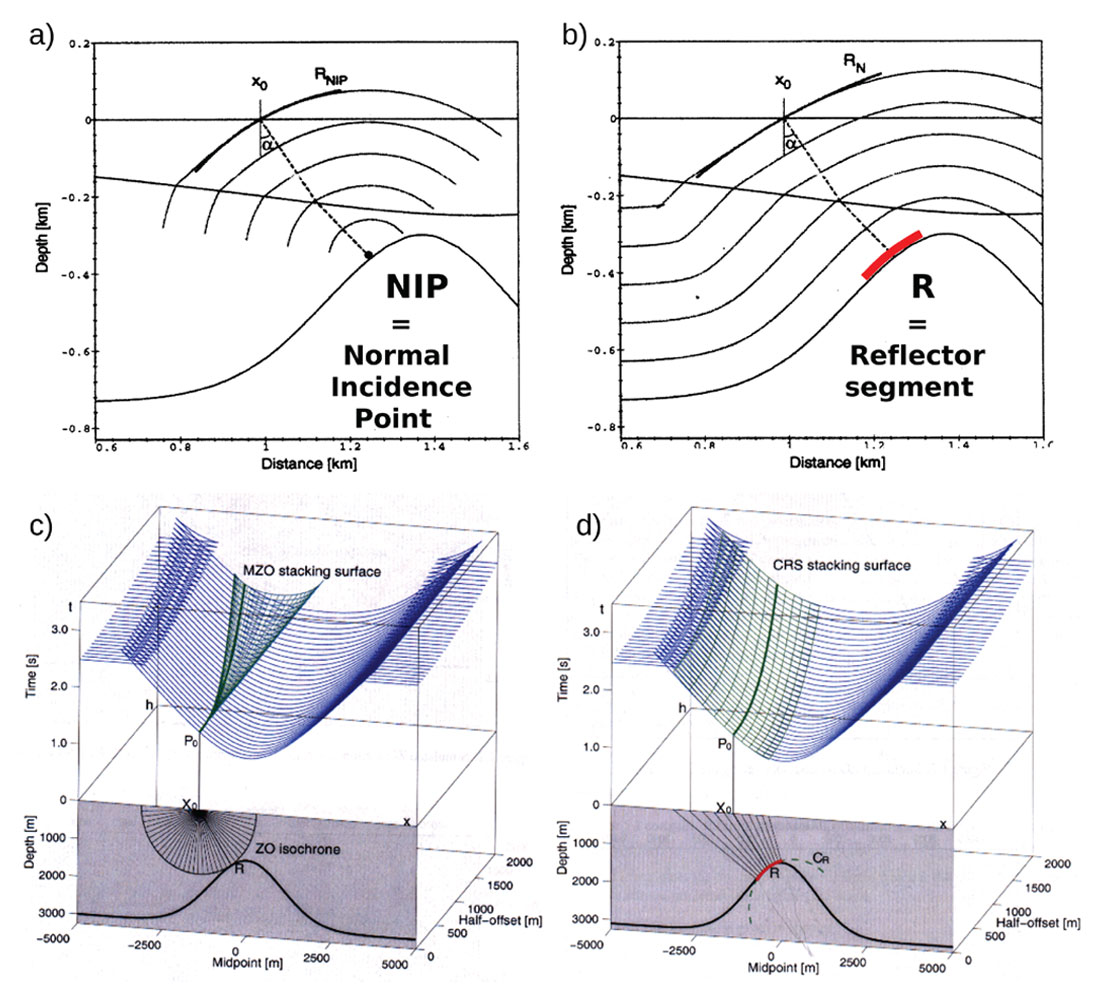
The excellent fit of the CRS stacking surface to a reflection event is illustrated by comparing conventional DMO stacking (c) to CRS stacking (d). For the anticlinal model in the lower part of the graphs, the common-offset travel time functions (blue) are displayed above for CMP location x and half-offset h. The DMO stacking surface (green) collects the reflection event (blue) only in a small tangential area along the solid green line (c), whereas CRS stacking (green) well follows the event in a large area (d, after Hubral et al. 1999).
With the CRS technique it is possible to reconstruct missing traces in a seismic data set by mapping seismic event data along the CRS traveltime surfaces. This interpolation can be used in any type of data regularization, or specifically in order to fill certain acquisition gaps. Unlike other interpolation algorithms (e.g. 5-D interpolation) CRS processing can handle data from complex geology and steep-dip reflectors (Fig. 2). The selected type of regularization depends on the intended processing and imaging sequence. For Kirchhoff prestack migration a regularization in CMP-offset domain is the preferred choice, whereas for depth migration in the shot domain by RTM a shot-receiver regularization is adequate.
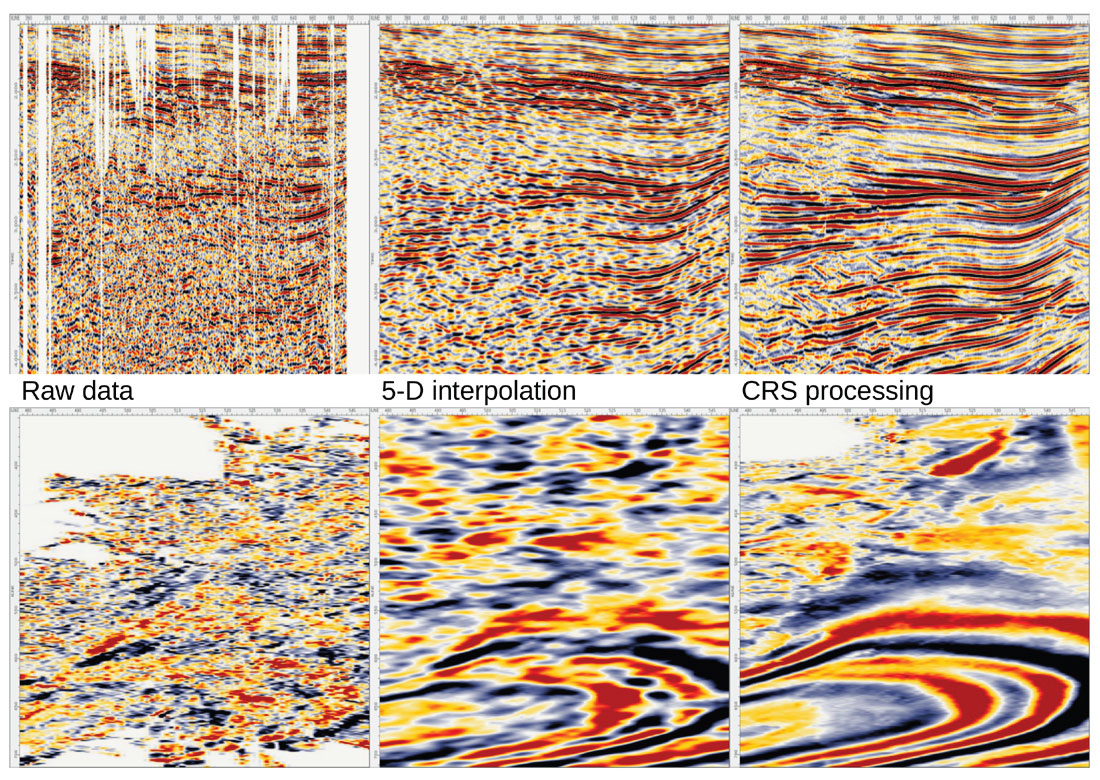
Alpine data set
The data set processed in this case history was acquired in the transition zone between the Northern Calcareous Alps and the Molasse Foreland Basin, Austria. The Molasse Foreland Basin covers a vast area north of the Alps, and deepens southwards towards the Alps. The Molasse sediments are divided in three sections: Tertiary Molasse, Imbricated Molasse, and Subalpine Molasse. The Imbricated Molasse is associated with the nappe tectonics of the Alps. These nappes were formed by the north-south oriented stress field, and thus are divided by mainly east-west striking faults.
Table 1. Acquisition parameters for the 3-D seismic data set, Northern Calcareous Alps |
source line spacing: 500 m |
source point spacing: 50 m |
receiver line spacing: 200 m |
receiver point spacing: 50 m |
receiver points per patch line: 120 |
symmetric split spread |
receiver lines per spread: 16 |
number of channels per spread: 1920 |
nominal coverage: 48 fold |
The seismic data was acquired in several 3-D surveys (from 1984 to 2004). The data examples shown here are taken from the latest data set, with parameters being summarized in table 1. Receiver lines are mainly oriented north-southwards with orthogonal shot lines to best image the east-west striking faults.
CRS processing and Kirchhoff migration
The initial processing approach comprised a general appraisal of the image improvement to be expected from the CRS method. A traditional CRS offset regularization significantly enhanced the signalto- noise ratio and the continuity of reflectors in the complicated thrust geology. In Fig. 3, the resolution of thrust planes and anticlines in time migration is superior by prior CRS data preconditioning, than by conventional time processing.
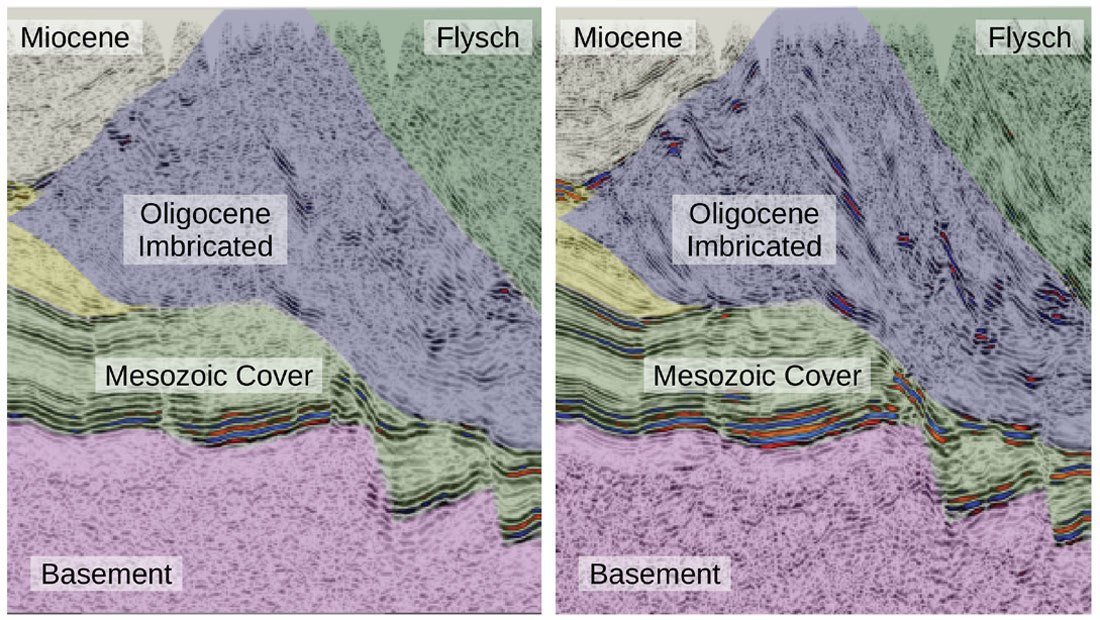
Departing from this initial CRS performance, the CRS technique was used to regularize the data in offset and azimuth domain, using equidistant azimuth sectors. Due to shot-receiver reciprocity the valid azimuth variation is reduced to the range of 0° – 180° which was covered by six azimuth classes of 30°. The decomposition of the original data into these azimuth classes obviously resulted in very low fold levels as an individual class comprises 5 to 10 traces on average (Fig. 4).
CRS regularization then largely reconstructed the missing traces from the available wavefield. In the azimuth sectors, the fold level was increased to a level between 20 and 40 (Fig. 4). However, not all azimuth sectors were completely filled: In the sectors bordering to 90° (east-west direction) only near-offset traces were available. This was due to the north-south layout of the acquisition.
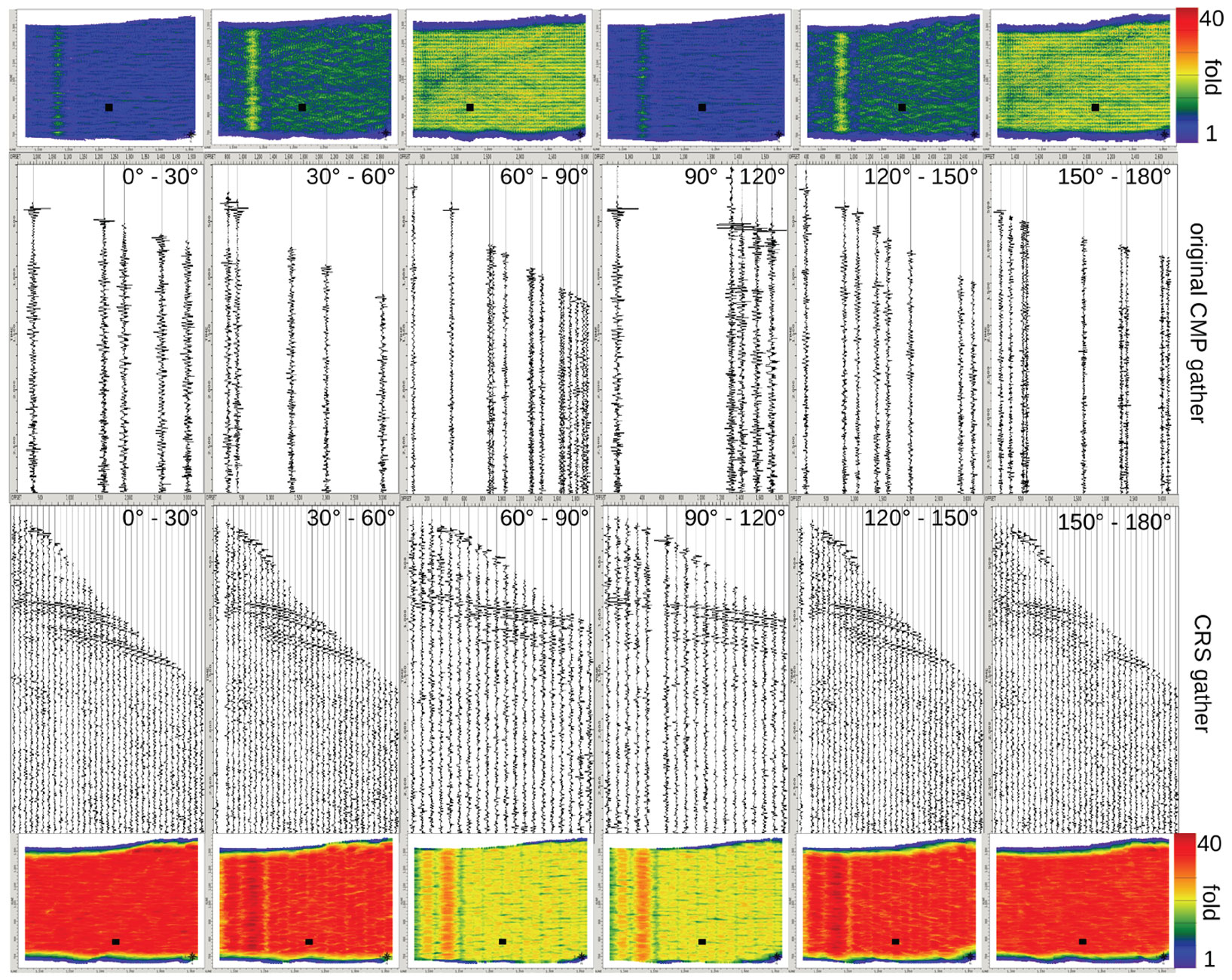
The well filled CRS azimuth gathers were then migrated separately using a Kirchhoff algorithm. Within each azimuth class different fault details and geologic features are visible (Fig. 5), which implicates a more detailed interpretation of fault and fracture orientation. Although the main fracture orientation is east-west, the azimuth classes near 90° emphasize some additional faults, which run north-south. In Fig. 5, a northern and a southern fault are interpreted in the zoomed sections of the single azimuth migrations. While the northern fault is visible on all azimuth classes, the southern fault vanishes in the two classes near 90°. This fault is either a small north-south trending feature, that only appears when illuminated at a right angle. It can also be a phantom fault, induced on the seismic data by the complicated geology overlying this region.
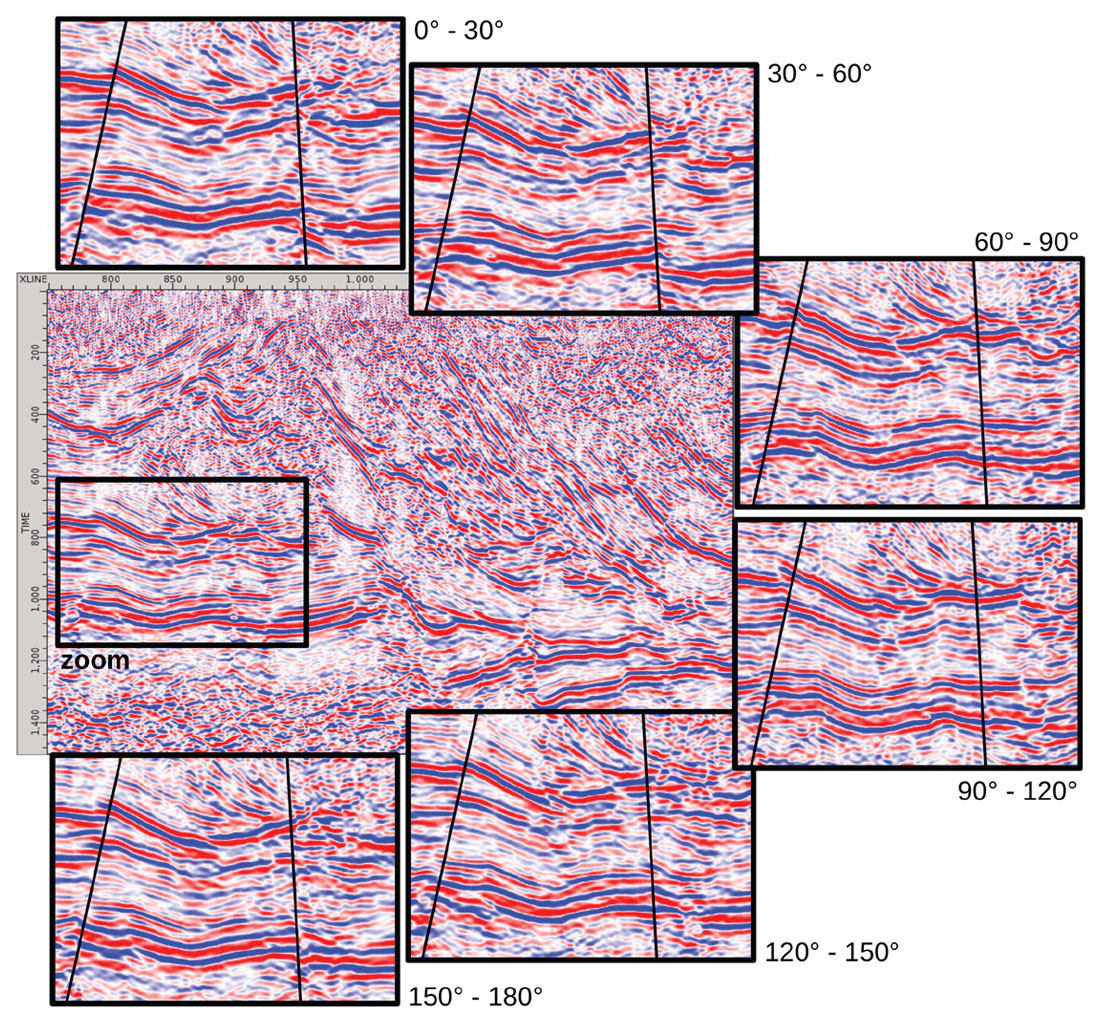
Reverse Time Migration
Reverse Time Migration (RTM) is an advanced depth migration method based on two-way wave equation modeling of source and receiver wavefields that are combined by an imaging condition. The imaging condition, commonly a wavefield correlation, transforms the modeled wavefields to the final depth image.
RTM is considered to be the most adequate migration algorithm for complex tectonic settings, which has been demonstrated in a wide range of applications. However, most of the case studies discussed in literature are marine data examples. One of the reasons for the absence of land data may be the reduced fold and low signal-to-noise ratio, often combined with irregular geometry. In this case study, the combination of CRS and RTM was tested on a complex land dataset as promising approach to overcome these disadvantages.
The RTM modeling used a pseudo-spectral code based on fast Fourier transformations (FFT) to solve the spacial derivatives of the seismic wavefield. This approach is more accurate than FD operators due to the large operator size: The FFT code processes the complete extension of the seismic model concurrently, while the FD approach only uses the neighboring samples.
Fig. 6 demonstrates the impact of CRS processing in combination with prestack depth migration using different algorithms. The Fig. 6a) and 6b) illustrate the use of conventional NMO gathers versus CRS offset-regularized gathers in Kirchhoff depth migration. Especially in the upper part of the central fault zone, the CRS processing increases the event continuity and resolution.
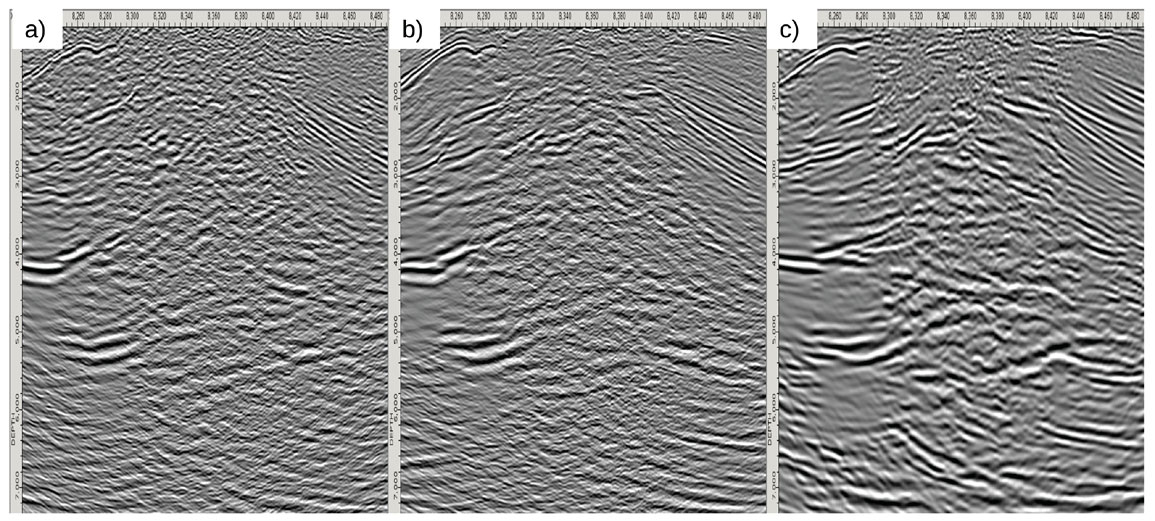
In order to reconstruct the deeper part of the fault zone from wavefield contributions with increasing complexity, we move from ray-based migration to wave-equation migration. Figs. 6b) and c) compare ray-based Kirchhoff depth migration of CRS offset-regularized gathers to RTM of CRS shot gathers. The CRS-based RTM image improves the depth resolution throughout the section, clarifying the main structural units and faults from shallow details down to large depths. Although the frequency content is lower than in Kirchhoff migration, the structural information is significantly improved in the RTM.
Conclusion
This case study applies a CRS processing as a well established technology to improve the continuity and overall signal-to-noise ratio of seismic reflection data. Due to its detailed parameterization of the entire wavefield, the CRS methodology additionally provides a flexible regularization and data reconstruction at acquisition gaps which is superior to a 5-D interpolation result. For different migration algorithms, different types of CRS regularization are demonstrated to solve the imaging and interpretation tasks.
A CRS regularization in the offset-azimuth domain is used to produce well populated azimuth classes that are subsequently input to individual Kirchhoff migrations. The major faults of the subsurface are resolved in all azimuth classes, whereas small, more subtle features are imaged in individual classes with more profound orthogonal illumination. This azimuthal regularization and imaging is thus providing both, more details of the subsurface geology, and additional confirmation on the orientation of small features.
A CRS regularization in the shot-receiver domain provides a uniform areal shot coverage as input to Reverse Time Migration (RTM). The regular CRS shot gathers minimize migration footprints in RTM imaging, and the enhanced signal-to-noise ratio of the CRS processing improves the seismic events in general. It thus provides a strong increase in resolution of the complex subsurface structures, and compensates for the typical irregularity issues of these low-fold seismic land data.
Join the Conversation
Interested in starting, or contributing to a conversation about an article or issue of the RECORDER? Join our CSEG LinkedIn Group.
Share This Article