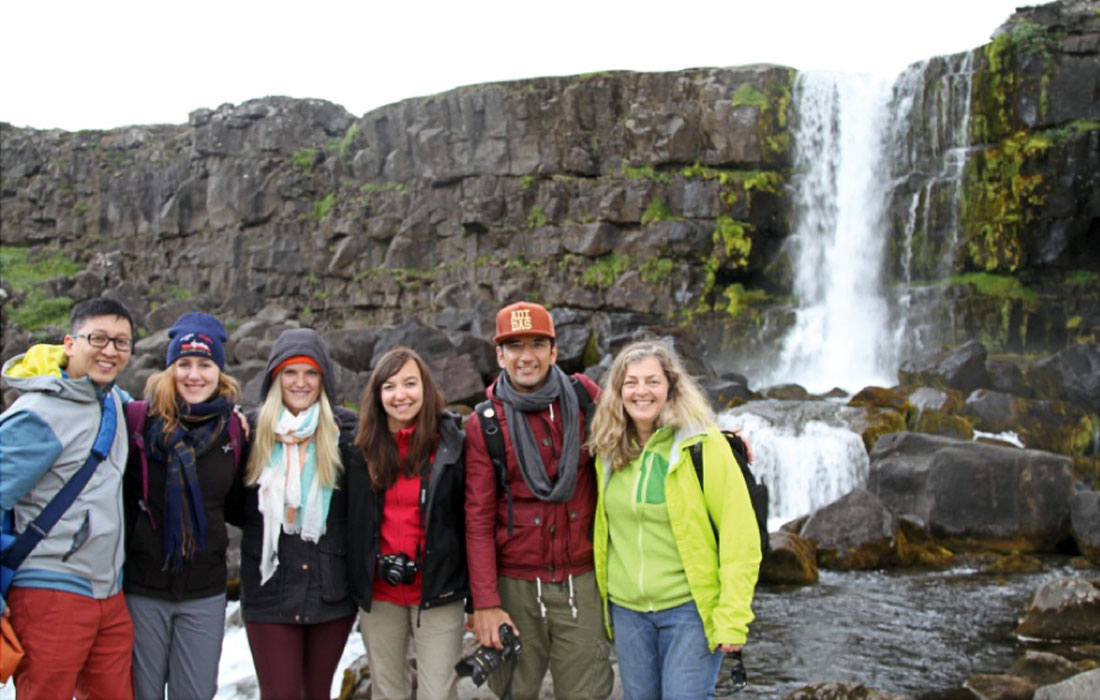
An 11 day field trip was undertaken to Iceland and Northern Norway to gain an understanding of past and present geomorphological and tectonic processes that lead to the unique landscape of Northern Norway, the processes that formed the continental and oceanic crust, and the geothermal and volcanic activity of Iceland.
Participants: Taylor Campbell, Anne Hargreaves, Rachel Milligan, Laura Broom, Carlos Wong and Masoud Ali
Introduction
Members of the Dawson Geology Graduate Society participated in an 11-day geological, field trip to Iceland and Northern Norway to learn about each region's unique geology. Students were able to enhance their geological knowledge through hands-on experience and exposure and had the opportunity to represent Dalhousie University abroad. Six Earth Science graduate students travelled to Iceland and Northern Norway from July 12-23, 2016. The first four days of the field trip were spent in Iceland, and for the remaining 6 days the students travelled around the Lofoten Islands and the mainland of Northern Norway. The following explains the geological significance of both regions, from the active spreading centre of Iceland to the tectonic and geomorphological processes that formed the unique landscape of the region within the Arctic Circle of Northern Norway.
Iceland
Iceland was the first stop of our trip, where we had 4 days, from July 13-July 17, 2016 to explore the unique and beautiful country. As geoscientists, experiencing Iceland first-hand is an invaluable experience. It gives a unique opportunity to view and envision what Earth was like early in its formation. It shows us the boundary of the Eurasian and the American tectonic plates with abundant glacial, fluvial and lacustrine sediments. The history of Iceland involves the interaction of both the fire of volcanoes and the ice of glaciers, during the deposition of alternating volcanic and sedimentary successions, which have been deposited subaerially, subglacially and subaqueously (Thordarson and Hoskuldsson, 2013).
Geology of Iceland
Iceland is the youngest section of the volcanic province stretching from Scotland to Greenland, and is located between 63 – 66 degrees latitude north. The Icelandic basaltic plateau rises 3 km from the seafloor and has an area of 350,000 km2. To give an idea of perspective, 70% of the landmass is below and 30% is above sea level, with an offshore shelf 50 – 200 km wide sloping to about 400 m in depth, and then dropping off. The island is a young country, formed during the last 25 Ma in the Tertiary and Quaternary, with the oldest surface rocks dating to 14 – 16 Ma. It has a high rate of erosion with estimated 1 million m3 eroded per year due to freeze, thaw, wind, seas, and glaciers, however sedimentation and volcanism add back likely as much (Thordarson and Hoskuldsson, 2013).
The rifting plate boundary is located over a hot spot, where a buoyant mantle plume causes a bulge in the crust, resulting in Iceland rising above the water level as a plume of hotter and less dense material is extruded. It is estimated that 10 million km2 of magma (or 50x the volume of Iceland's existing land mass) has been extruded over the last 65 Ma. Submarine Faeroe – Iceland (towards the SE) and Greenland – Iceland ridges (towards the NW) are older traces of this bulge now displaced by seafloor spreading. Modelling indicates a 200 – 300 km wide cylindrical zone of viscous semi-solid material, which is hot and buoyant and rising slowly from 400 – 700 km depth. As it nears surface, it provides melt. At 24 Ma, the mantle plume was at intersection of the Kolbeinsey spreading ridge in the north, and the Reykjanes spreading ridge in the south (see Figure 1).
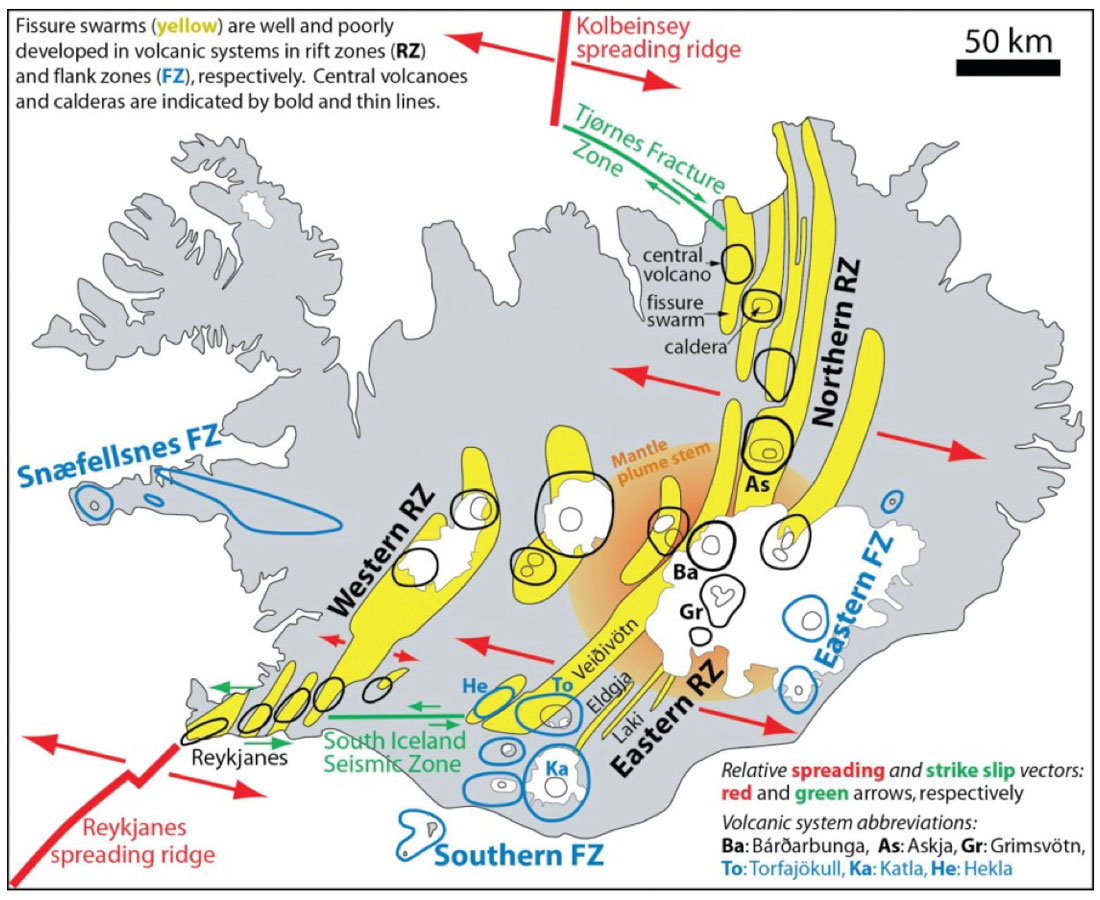
There is a narrow belt of active faulting and volcanism, which runs from Reykjanes peninsula (SW by airport) which zig zags to Oxarfjordur, at the tip of the Northern RZ (rift zone) (see Figure 1). The volcanic zones are 20 – 50 km wide, and the centre of the zones accumulate the most magma, which sinks rapidly and causes down-sagging of the crust, which causes it to tilt 5 – 10 degrees towards the axis. Shallow synclines are formed resulting in layers dipping towards volcanically active zones.
The relative positions of the spreading axis and mantle plume have changed over time. If the mantle plume is assumed to be stationary, the spreading axis has migrated WNW at 0.3 cm per year concurrently with the spreading motion separating the plates (Thordarson and Hoskuldsson 2013). The plume basically readjusts the position of the spreading axis as the axis moves away, which results in what is called a ‘rift jump’. This rift jump results in trapped sediments and older crust between the two zones (WRZ and ERZ) causing sagging, which results in synclines and anticlines across the area (see Figure 1).
Two active intraplate volcanic belts with young (less than 2 Ma rocks) are unconformably over top of older formations, pointing to a major magmatic episode. One of these we will be able to visit on our trip, which is the Snaefellsnes Volcanic Belt. It is located in west Iceland sitting on the mantle plume trail. (See Figure 1, labelled Snaefellsnes FZ (flank zone).
“Golden Triangle” Tour
Our day spent viewing the “Golden Triangle” took us to Þingvellir, Geysir and Gullfoss. Southern Iceland is located in the area between the West Volcanic Zone and the East Volcanic Zone (see Figure 1). This area is where the largest earthquakes in Iceland occur, generally between 6 – 8 on the Richter scale; most recently in 2000 and 2006. Here, there are N – S trending strike-slip faults with E-W trending transform faults linking the WVZ and the EVZ (See Figure 1). The last ice age (Weichselian) retreated from here about 10,000 years ago, leaving the area underwater. Due to isostatic rebound, it emerged during the Holocene becoming a vast flat coastal plain. Also during the Holocene, a series of at least 8 huge fissure-fed basalt lava flows occurred in the region, which indicates that the production rate of basalt during that time was greater than today. This has been attributed to rapid crustal rebound due to the removal of up to 1000 m of ice (Thordarson and Hoskuldsson, 2013).
Þingvellir
Þingvellir is famous for being the cultural site of the Icelandic open air parliament held since the 930 AD. The site is on the edge of a fault-bounded lake basin, the Þingvellir graben, which has a basement of Holocene basalt lava flows. The graben is surrounded on all sides by four active volcanic systems, which produced mostly pahoehoe lavas, creating the cliffy scenery that we walked along at the UNESCO World Heritage Site (see photo above). The Þingvellir graben is thought to be the surface expression of deeply rooted normal faults which extend deep into the crust and formed as a result of sea floor spreading. During the past 10,500 years, the graben floor has subsided at least 40 m, or 1 mm per year, and extended horizontally 70 m or 7 mm per year. The rifting occurs episodically however, with changes of, for example, 3 m at a time. After such a drop in 1789, the parliament’s annual assembly at Þingvellir was move to Reykjavik (Thordarson and Hoskuldsson, 2013).
Geysir
The place name of Geysir gave the geological phenomenon of spouting hot springs their name, universally recognized the world over. The waters here are alkaline with clear water, and located in an area of extensive siliceous sinter deposits. These are created as silica rises to the surface dissolved in superheated water. Upon cooling, it forms a deposit referred to as silica sinter (Lynne, 2013).
Geysir itself is a 3 m wide and 22 m deep channel which changes to a 15 m wide and 2 m deep bowl at the surface, which is referred to as a sinter dome. The water is superheated at a depth of about 10 m as the surface water, initially flat and quiet, starts to bubble and swirl, then rises up into a cupola of turquoise colour (see below), and then erupts into water jets up to a height of 100 m or so. We were able to observe the Strokker geyser, which erupts every 5 minutes or so. Water temperature in this area is 80 – 100 degrees C.
Gullfoss
This spectacular waterfall (Figure 2) occurs where the River Hvita flows from the highlands and enters the Southern Lowlands, eroding into an impressive gorge 2500 m long and 70 m deep. The river route itself is fault controlled and contrasting lithologies of rock create the gorge. A succession of thick, clastic layers is capped by thinner lava layers. The sedimentary layers are undercut, as they erode much easier, leaving the thinner resistant lavas to overhang and support the water. These overhangs periodically collapse and the process repeats itself. This gorge is approximately 10,700 years old, with the gorge being carved out at about 25 cm per year. Catastrophic floods, called jökulhlaups, which occurred as the Younger Dryas glacier retreated from 11,700 – 10,500 years ago, may have contributed to its formation (Thordarson and Hoskuldsson, 2013).
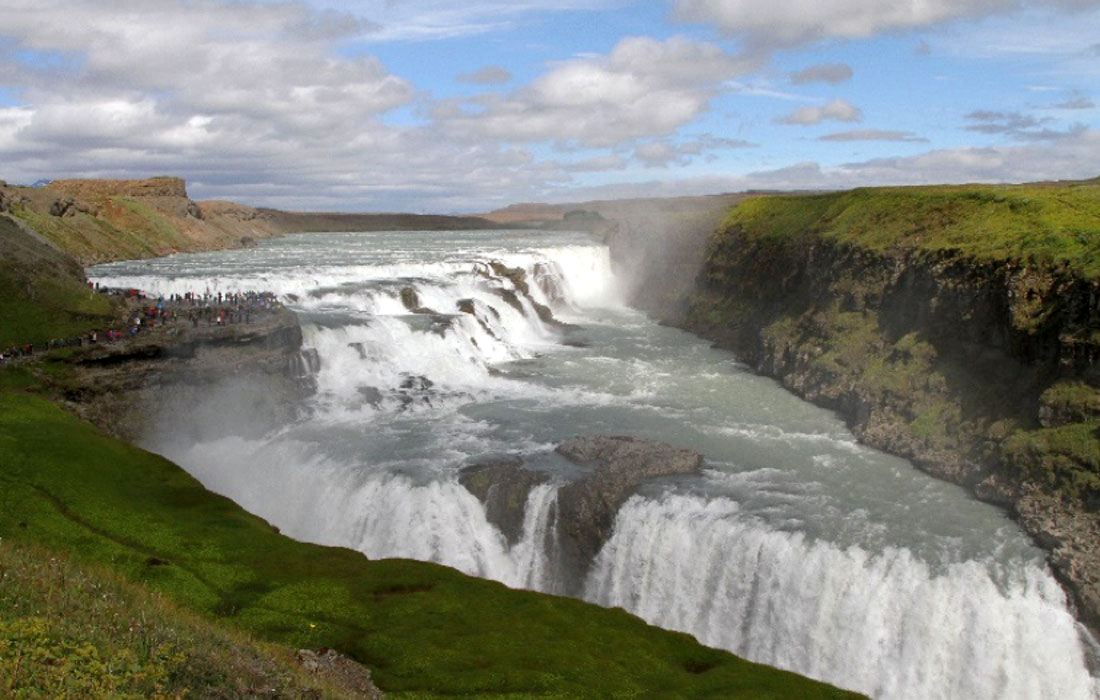
Eyjafjallajökull volcano
Eyjafjallajökull has produced some 14 – 16 eruptions since the last ice age. Most recently, in 1994 and 1999 seismic swarms lasting a month occurred under Eyjafjallajökull, which indicated magma injections of sills between the volcano and the crust. Surface deformation of Eyjafjallajökull was seen from satellite images, and in 2009 another seismic swarm plus further surface deformation occurred. On the 20th of March 2010 a 400 m fissure opened up on Eyjafjallajökull with fire and 150 m high lava fountains creating a basaltic a’a lava flow until April 12. Next on the 14th April, a trachyandesite of intermediate composition began to shoot out of the summit of Eyjafjallajökull cutting through 250 m of ice, and causing glacial meltwater to cover the sandur plain seen from the Seljalandsfoss waterfall. Steam changed to tephra (ash, lapilli and bombs) rising 8 km in the air. This carried on until the 22nd of May, ultimately producing 323 million m3 of material and interrupting international airspace (Thordarson and Hoskuldsson, 2013).
Snæfellsnes
The Snæfellsnes peninsula is 10 to 30 km wide and extends 80 km or so, at the end of which is the Snæfellsjökull stratovolcano (Figure 3). The Snæfellsnes Volcanic Belt traces the trail of the mantle plume as plate tectonics and sea floor spreading caused the plates to move. This trail traces volcanic activity from the mantle plume from the last million years only. These volcanic belts trend W-NW. (see the blue oblongs of the SFZ in Figure 1). We drove a circular route around this peninsula and were able to view Snæfellsjökull from all angles and cross the basaltic lava fields. This volcano has not erupted in human recorded history (Thordarson and Hoskuldsson, 2013).
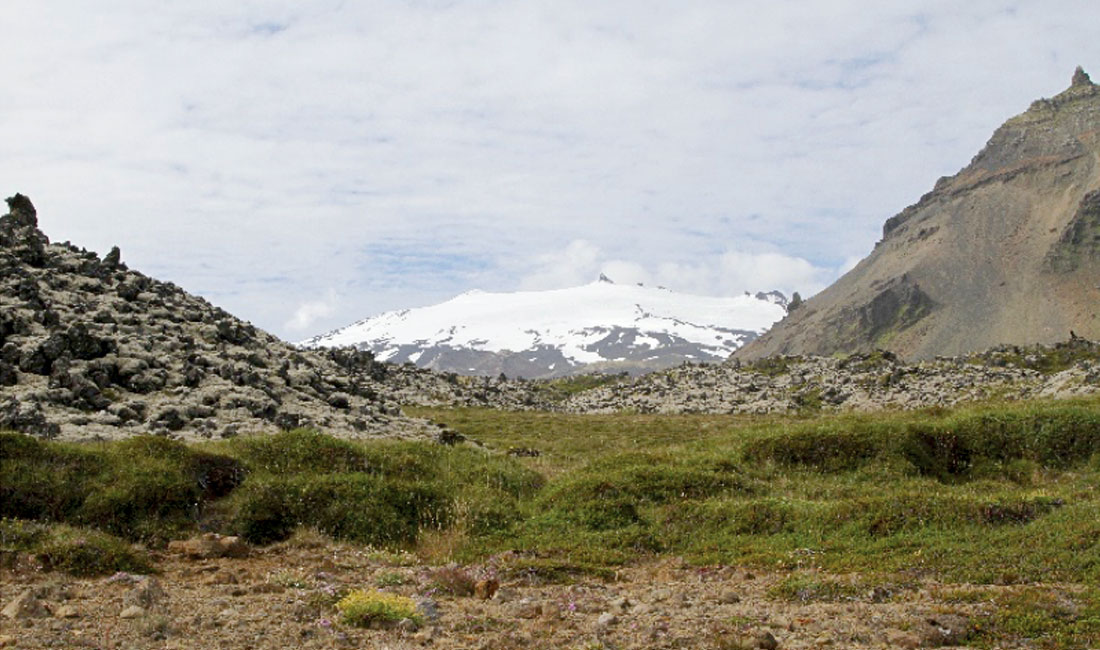
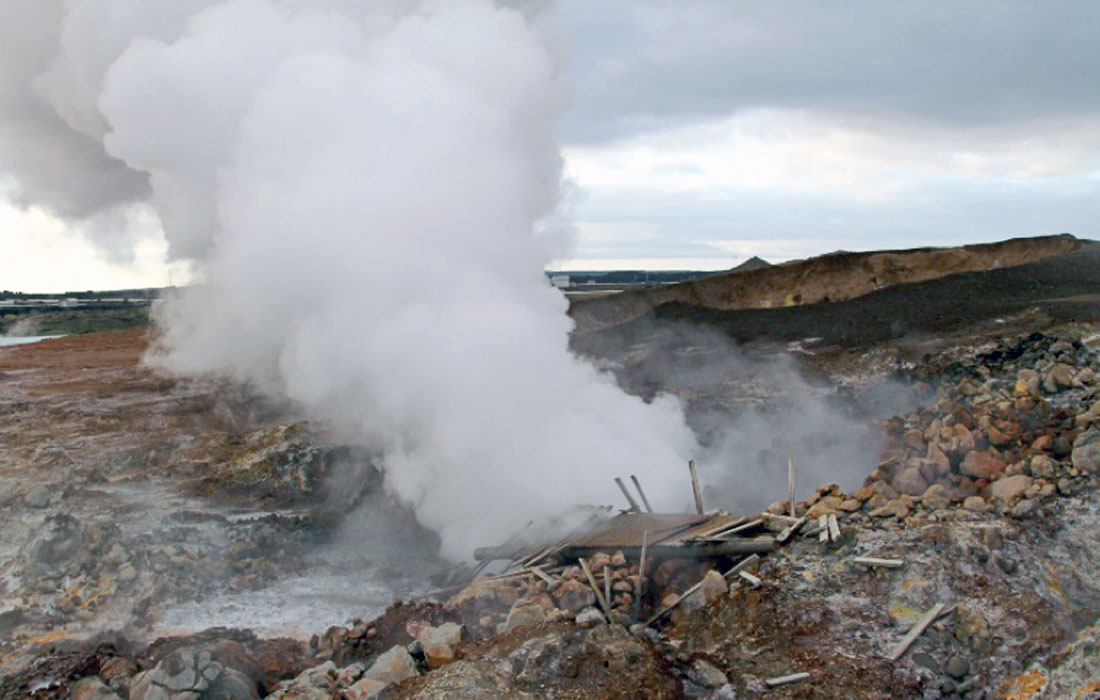
Reykjanes Peninsula
Intense volcanic activity has taken place since the last ice age. It is underlain by various fissure swarms which have been periodically active, the last major one being in 1926. Associated pahoehoe lava fields resulting from eruptions cover the landscape from the airport to Reykjavik. Further down the peninsula, south of the airport, we saw some fantastic steam vents.
Pillow lava examples are also seen. As magma reaches the surface underneath a glacier, a cavity melts in the ice. Water pressure holds the magma in ‘pillow’ shapes as it extrudes in the water. If water pressure holds, large pillow ridges form (Norðdahl et al. 2008).
Geothermal activity on Iceland
One of the most exciting geological features we saw in Iceland was the highly active Geysir Hot Spring (Figure 5). The geysers in this area could spout boiling water up to 100 ft into the air every a few minutes which shows high geothermal gradient underneath the spring. Having hundreds of geothermal hot spring similar to Geysir all over the country. Iceland provides more than 80% of its energy using the geothermal and hydroelectric resources (Figure 6). By drilling deep wells (~ 2.5 – 5 km) in areas with extremely high thermal gradient, tapping a supercritical reservoir at temperatures above 450°C, Iceland provides about ~50 MWe per well. Moreover, a consortium of the Icelandic government, the U.S. National Science Foundation, the European Union and Alcoa have banded together on the Iceland Deep Drilling Project (IDDP) to study high-temperature hydrothermal systems in Iceland. Over the next few years the IDDP is drilling and testing a series of boreholes to penetrate supercritical zones underneath the currently exploited geothermal fields in Iceland.
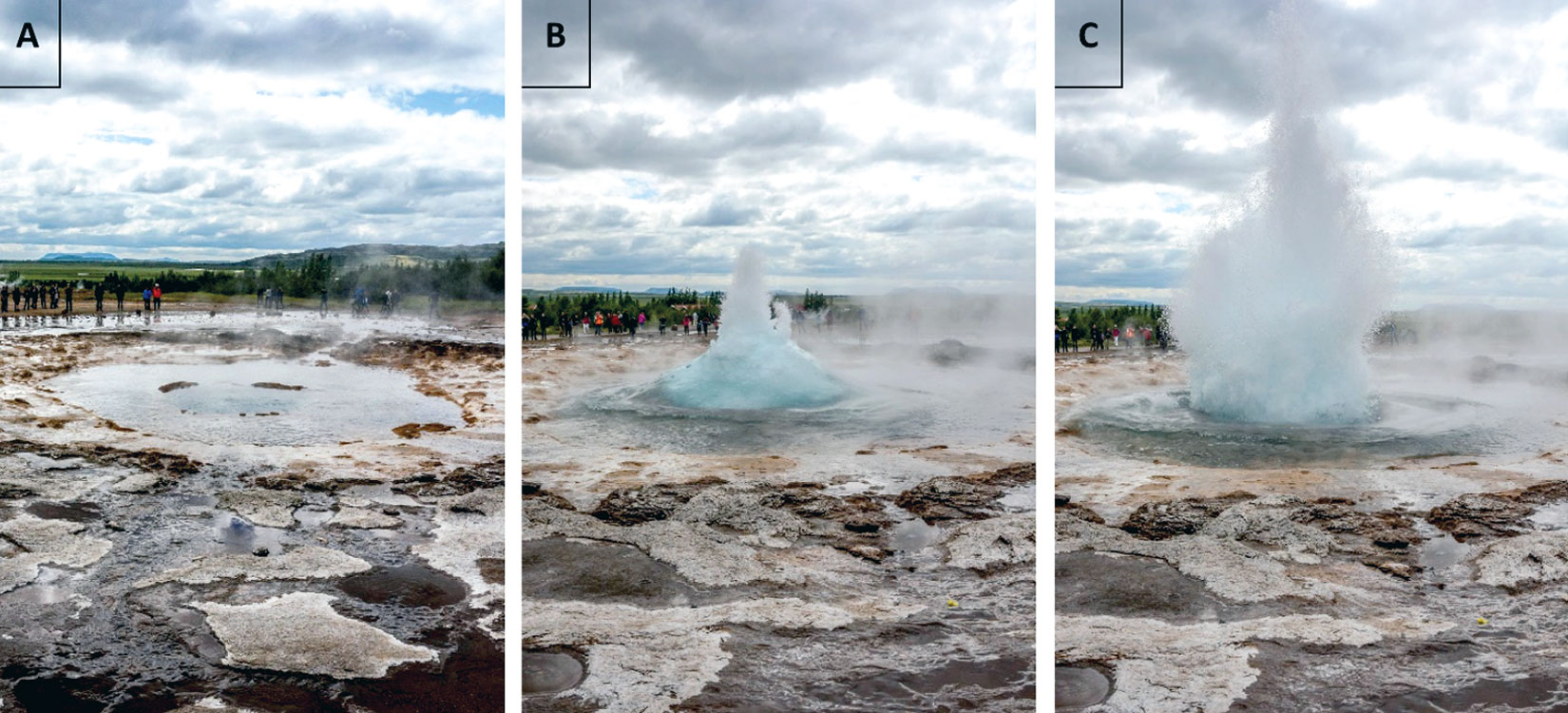
Geophysical method used in geothermal exploration
Geophysical methods are one of the main disciplines used for exploring geothermal resources and characterising their properties. The exploring methods are useful to determine the geological structure of the environment and the parameters influenced by the geothermal activity, such as thermal gradient and rock resistivity. For instance, determining the structural features of volcanic and hydrothermal systems can be used to infer the location of magma chambers or productive geothermal areas (Jousset et al., 2011). Other geophysical methods used for geothermal reservoir characterisation measure the physical parameters of the host rock, including fracture density, seismic velocity, and magnetic properties. Thermal methods, electrical methods, and Transient Electro-Magnetic are common methods used for characterization of geothermal reservoirs (Figure 10).
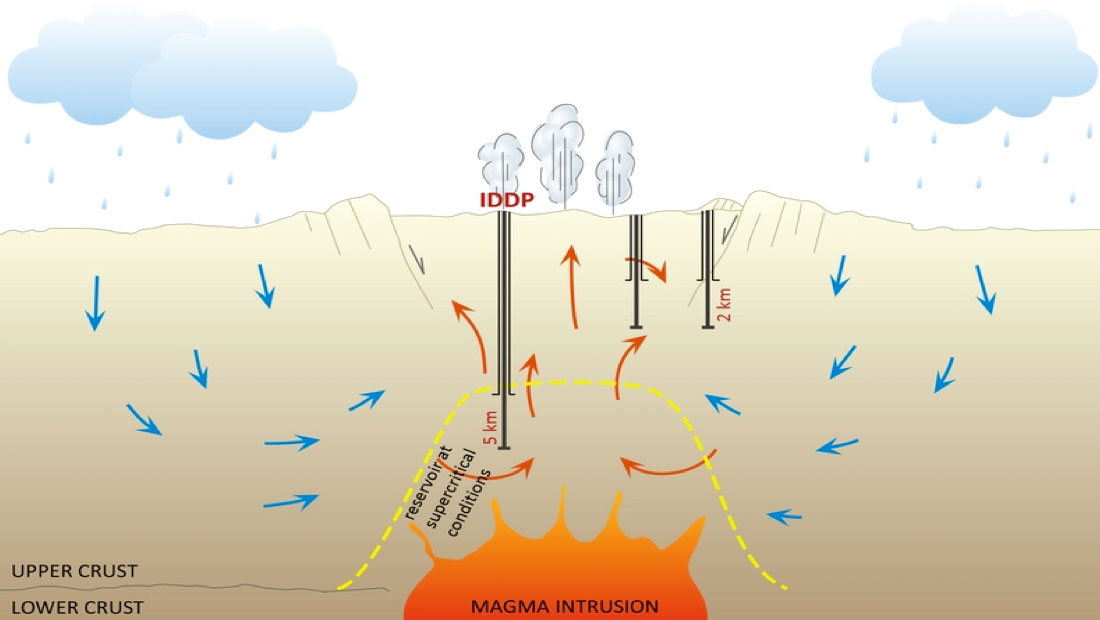
Lofoten Islands, Northern Norway
Lofoten Islands, located in Northern Norway within the Arctic Circle, are known for a distinctive scenery with dramatic mountains and peaks, open sea and fjords, as well as world class beaches. Our group was fortunate to visit three of the eight main islands of Lofoten on July 18-22, 2016 (Figure 7). The islands we visited included: Moskenesøy, Flakstadøy and Vestvagøy. We also were able to see the islands of Gimsøy, Austvågøy and Hadseløy on our drive to Harstad, Northern Norway. To get to the Lofoten Islands we took a ferry (3.5 hours) from Bodø to Moskenes. We then drove from Moskenes to Vestvagøy, where along the way got an initial view at the mountainous landscape that made up the Lofoten Islands.
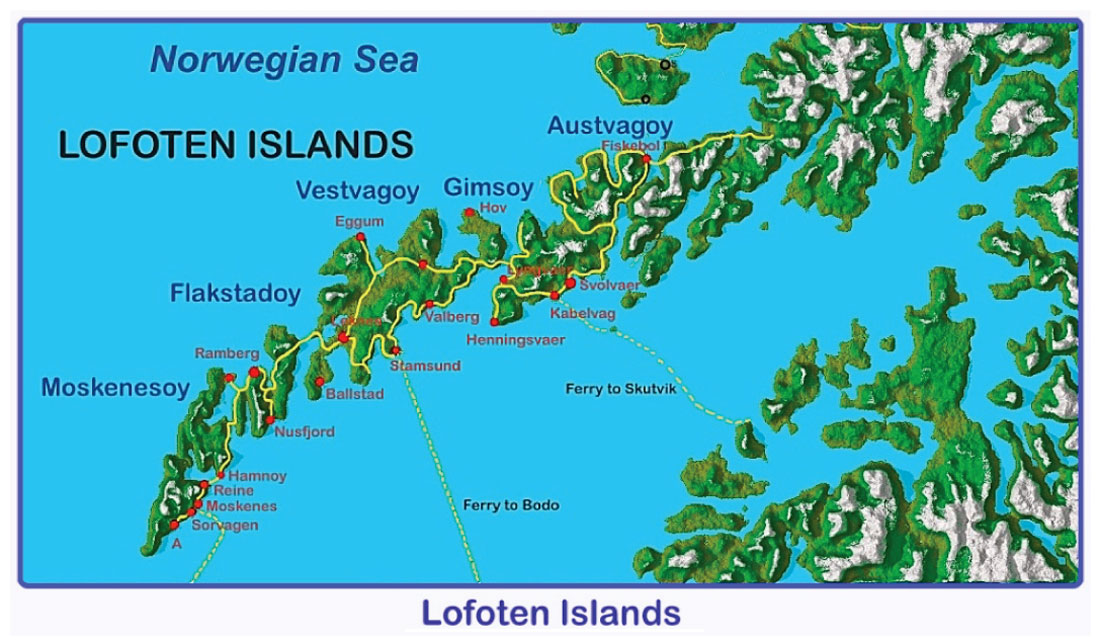
The Caledonian Event
The oldest rocks within the Arctic Circle region were formed in the Precambrian, approximately 1.7-1.8 Ga. Within Norway, they are referred to as “grunnfjell” which means basement in Norwegian. These rocks later formed the basement for the Caledonian mountain chain, developed in the Paleozoic (Bargel, 2009). Towards the end of the Precambrian, the North American and Eurasian plates were pushed away from one another due to rising magma, which rose between fractures and became solidified, forming new ocean floor. This process led to the formation of the Lapetus Sea. In the Later Palaezoic, the Lapetus Sea began to close when these two continental plates were pushed together, folding and thrusting over the older basement and gradually building the mountain chain consisting of thick sequences of thrust slices and nappes (Bargel, 2009). During the thrusting and folding, the rocks underwent metamorphism, resulting in schists and marbles. The collision also allowed for magma to intrude and solidify as an intrusive rock. The final phase of folding and thrusting took place during the Devonian, which marked the last development stage of the Caledonian mountain chain.
The Caledonian mountain belt extends through western Scandinavia. The Caledonian orogeny took place during the Silurian and Early Devonian, when there was a continent-continent collision between Laurentia (Greenland and North America) and Eurasia (400-450 Ma). Local eclogites in shear zones and pegmatites are the only product of the Caledonian orogeny in the Lofoten islands (Bergh and Corner, 2015). Eclogite-facies rocks with relics of omphacite as well as garnet as main minerals occur at five localities on two of the islands (Flakstadøy, Austvågøy). This indicates the region underwent a Caledonian high-pressure event, which Markl and Bucker (1997) had concluded was most likely related to Mesoproterozoic orogenic activity (Bergh and Corner, 2015). The rocks exposed at surface today were situated at considerable depth in the crust (approximately 45 km).
Geology of Lofoten Islands
The basement rock of the Lofoten islands is dominated by Archean and Paleoproterozoic para- and orthogneisses (granulite and amphibolite facies) and anorthosite-mangerite-charnockite-granite suites (Markl, 2001). Charnockite describes a unique mineral assemblage of a felsic magmatic rock (commonly in the monzonite compositional range) with pyroxene. This term can be applied to igneous charnockitic rocks crystallized directly from a melt, or to meta-igneous rocks which achieved charnockite character through metamorphism(Emslie and Hunt, 1990). The charnockite outcropping in the Lofoten islands, Norway, is of the first variety, occurring alongside anorthosite massifs, which has significant indications for the petrogenesis of AMCG suites and their relation to collisional orogenies.
The presence of magmatic charnockite suites in association with granulite-grade metamorphism has important implications for high-temperature gradients in the crust. Though the debate continues regarding the generation and nature of cogenetic metamorphic and magmatic materials, the occurrence of igneous anorthosite-mangerite- charnockite-granite (AMCG) suites accompanying granulite metamorphic terranes is seen to conform with unusually high temperatures in the deep crust (Emslie and Hunt, 1990). This phenomenon has been recognized world-wide in relation to large-scale orogenies, most notably, the Grenville event (1.10-1.03 Ga), including the Lofoten islands, in northwestern Norway (Emslie and Hunt, 1990; McLelland et al. 2010).
The current working hypothesis for the generation of high-temperature felsic igneous bodies in the crust involves over-thickened lithosphere, delamination, and multiple stages of magmatic fractionation (Markl, 2001; Martin, 2012; McLelland et al. 2010). Post-collision delamination of the lower lithosphere results in asthenospheric upwelling, and subsequent melting and degassing of mantle material. Crystallization and settling of olivine and pyroxene, accompanied by rising of buoyant plagioclase, results in segregated fractionates, with the feldspars sitting at the base of the crust. Residual crystallization heat melts the crust, and further fractionation of gabbroic material results in a residuum of mangerite, charnockite and A-type granite (Martin, 2012; McLelland et al. 2010).
The rarity of igneous rocks with charnockitic character and the association with granulite-facies metamorphism in the Lofoten islands, Norway, make it a unique place for study. Recent developments in modelling these relationships are important for understanding the tectonics and progressive evolution of large-scale thick-crust orogenies, such as the Grenville. Canadian Grenville terrane contains charnockite rocks of both igneous and metamorphic petrogenesis (Emslie and Hunt 1990). Direct field observation and recognition of these unique rock suites are the first step to understanding the deep crustal processes that derive them and the progression of orogenies that build continents. We had the opportunity to observe the relationship between charnockite suites and granulite gneisses in a well exposed and well-studied region, which gave us a distinct advantage when approaching geologic problems related to thick-crust orogenies in Canadian Grenville terranes. As well, the trip to Lofoten islands provided us an occasion to consider a complex multi-stage fractionation problem; a rare insight into the generation of felsic magma and the evolution of crustal material.
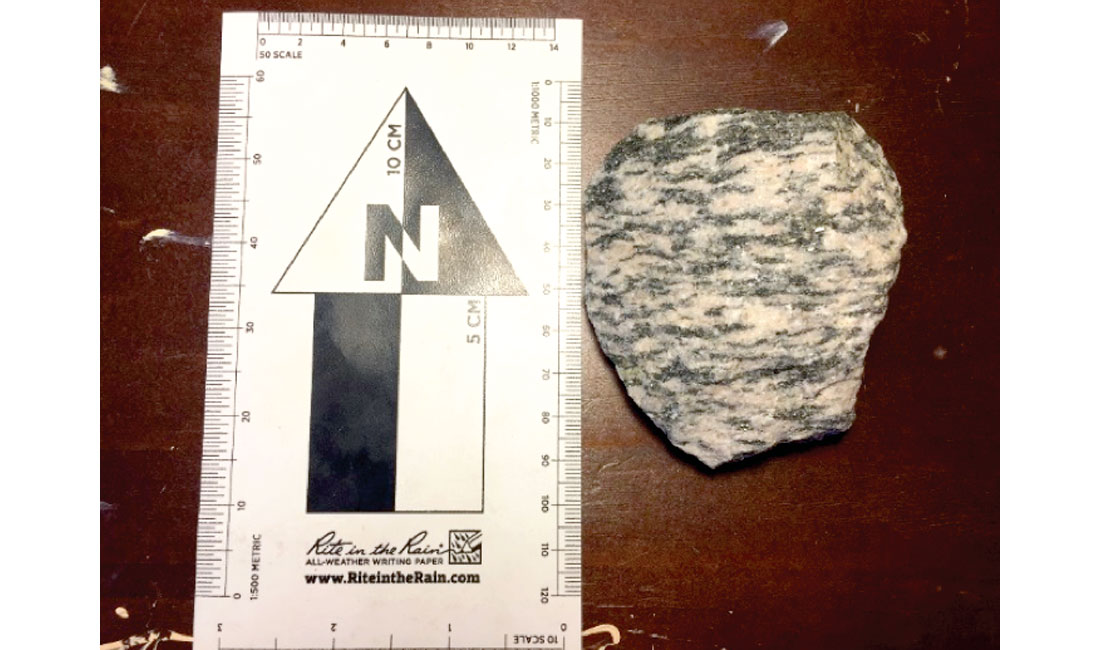
Share This CSEG Report