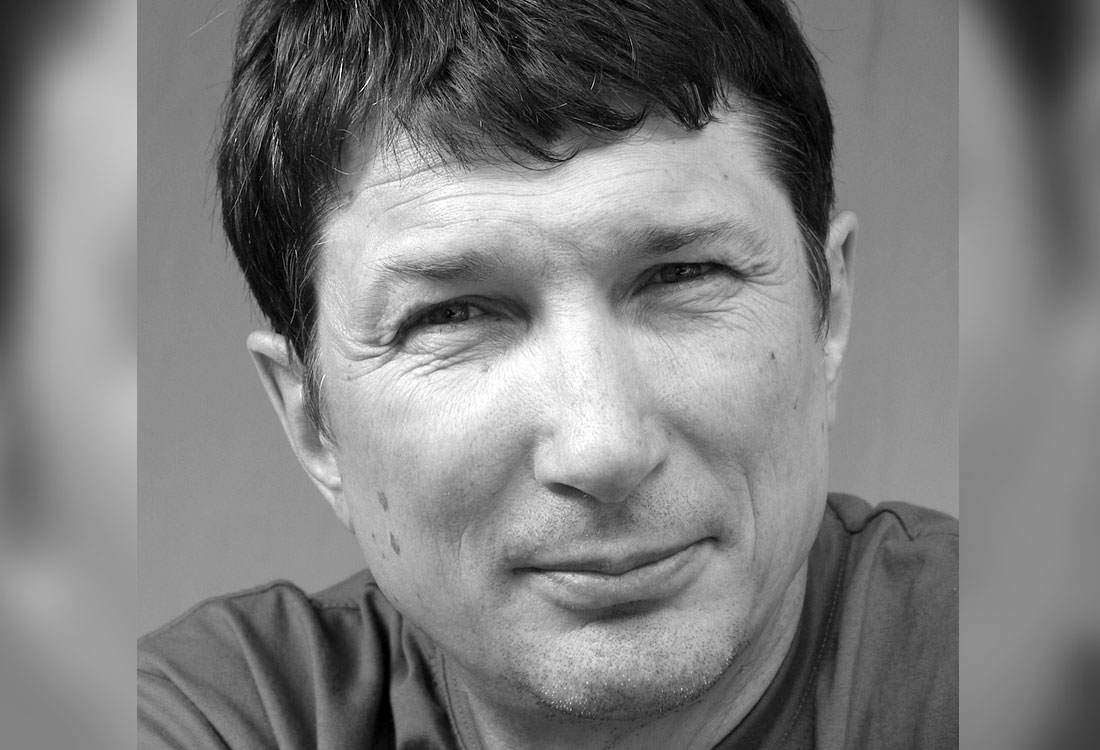
Taras Gerya is a professor at the Swiss Federal Institute of Technology (ETH-Zurich) working in the field of numerical modelling of geodynamic and planetary processes. He earned a Ph.D. in petrology from Moscow State University in 1990 and a habilitation in geodynamics from ETH-Zurich in 2008. His recent research interests include modelling of subduction and collision processes, ridge-transform oceanic spreading patterns, intrusion emplacement into the crust, and core formation of terrestrial planets. He is the author of Introduction to Numerical Geodynamic Modelling (Cambridge University Press, 2009). He can be reached at taras.gerya@erdw.ethz.ch.
I am a geodynamicist. Geodynamics aims to understand the evolution of the earth’s interior and surface over time. The following simple exercise explores the subject of geodynamics in the context of the availability of data. There are many parallels with modelling systems in exploration geophysics.
Let’s imagine an ideally symmetrical earth with physical properties (density, viscosity, temperature, etc.) as functions of depth and time. A simple two-dimensional time-depth diagram covering the earth’s entire history and its interior will thus be a schematic representation for the subject of geodynamics. For the real earth such a diagram should be four-dimensional, but this rectangular diagram will do for us. The entire diagram should be then covered by data points characterizing the physical state of the earth at different depths, ranging from 0 to 6000 km, and for different moments of geological time, ranging from 0 to around 4.5 billion years ago. However, the unfortunate fact for geodynamics is that observations for such systematic coverage are only available along the two axes of the diagram: geophysical data for the present-day earth structure and historical record in rocks formed close (typically within a few tens of kilometres) to the earth’s surface. the rest of the diagram is thus fundamentally devoid of observational data, so we have to rely on something else.
What else can we use? Scientific intuition based on geological experience and modelling based on fundamental laws of continuum mechanics! However, our intuition cannot always be suitable for geodynamical processes that are completely out of human scales in time (a few years) and space (a few metres). We have to accept that some of these processes could look completely counterintuitive to us. For example, acceptance of plate tectonics was delayed by relying on commonsense logic: a solid mantle conducting shear seismic waves can only deform elastically not allowing for the movement of continents over thousands of kilometres. The crucial point that was finally understood by the scientific community is that both viscous (fluid-like) and elastic (solid-like) behaviour are characteristic of the earth, depending on the time scale of deformation. The mantle, which is elastic on a human time scale, is viscous on geological time scales (>10,000 years) and can be strongly internally deformed by very slow solid-state creep.
The ways in which various geodynamic processes interact with each other can also be very difficult to conceive using only scientific intuition. This is why intuition in geodynamics should be – must be – assisted by modelling. In a way, modelling helps train our intuition for very deep and very slow geological processes that cannot be observed directly.
Another role of modelling is the quantification of geodynamic processes based on the sparse array of available observations. Consequently, the systematic use of both analog and numerical modelling is crucial to develop, test, and quantify geodynamic hypotheses – and perhaps most questions about the earth.
Q & A
Could you give us a picture of the evolution of earth’s interior briefly?
The evolution of the Earth’s interior is driven by two main processes affecting mantle circulation: (i) subduction of cold negatively buoyant oceanic plates lubricated by oceanic water and sinking from the Earth’s surface to the transition zone and core-mantle boundary (CMB) and (ii) upward propagation of hot positively buoyant mantle plumes generated at the core-mantle boundary around two anti-podal large low shear velocity provinces (LLSVP) presumably composed of chemically distinct dense mantle rocks of some primordial origin.
The plate tectonic theory proposed by Alfred Wegner was initially rejected by furious geologists, though he suggested many good reasons as to why continents/ plates appeared to move. Could you give us a more precise explanation for this rather beyond your general comments on the viscous and elastic behavior of the earth’s interior?
Initial criticism of Wegener’s theory was mainly physically grounded and concerned insufficiency of tidal/rotational forces (proposed by Wegener) to drive continental plate movements. This objection was overcome by proposing convective forces as the driver of plate movement. Later criticism was based on the apparent absence of physical deformation mechanisms within the solid elastic mantle (the solid state of the mantle was known due to its ability to transmit shear seismic waves), which would allow large horizontal continental motions. This objection was overcome by the discovery of solid-state creep, which allows viscous-like deformation of solid crystalline rocks on geological timescales.
In fact, Wegener’s theory had both supporters and critics. The short history would look like the following (partly subjective views given in my 2010 textbook):
1910: Frank B. Taylor, Continental Drift hypothesis. The real beginning of ‘drifting’ toward plate tectonics, still a long way to go.
1912–1946: Alfred Wegener, further developed the Continental Drift hypothesis, and showed a correspondence of the geological provinces, relict mountain ranges and fossil types. Driving forces – tidal/rotation of the Earth. Single protocontinent – Pangea. The principal questions are considered to be, ‘Why do continents move?’ and ‘What are the driving forces?’ and not yet, ‘How do continents move?’ and ‘What is the movement mechanism?’
1916: Gustaaf Adolf Frederik Molengraaff proposed mid-ocean ridges to be formed by seafloor spreading as the result of the movement of continents which in turn accounted for the opening of the Atlantic Ocean as well as the East Africa Rift. The mid-ocean ridges were ‘re-discovered’ for plate tectonics 40 years later...
1924: Harold Jeffreys showed the insufficiency of Wegener’s forces to move continents. Computing forces for testing a geodynamic hypothesis is one of the core principles of modern geodynamics as well! Another point to learn – opposition to the Continental Drift hypothesis using physical arguments was always strong and probably considerably delayed the theory of plate tectonics.
1931: Arthur Holmes suggested that thermal convection in the Earth’s mantle can drive continental drift. This crucial idea answered a question about driving forces, but not about the movement mechanisms. It was known from seismic studies, that the Earth’s mantle is in a solid state and elastic deformation does not allow thousands of kilometres of motion of the continents.
1935: N. A. Haskell established the fluid-like behaviour of the mantle (viscosity 1020 Pa s) based on the analysis of beach terraces in Scandinavia and the existence of post-glaciation rebound. Actually, this was also established earlier from inferring crustal roots. The question about the physical mechanisms of solid-state mantle deformation remains open.
1937: Alexander du Toit suggested the existence of two proto continents – Laurasia and Gondwanaland, separated by the Tethys Ocean. This is a really dramatic story: “geologists” were continuously developing and supporting the Continental Drift hypothesis, but the general idea of large lateral displacements of continents was continuously rejected by “geophysicists”.
1950s: Improved understanding of the worldwide network of mid-ocean ridges during extensive exploration of the seafloor. Evidence is critically growing in line with Molengraaff’s ideas...
1950s: Finding mechanisms of solid-state creep of crystalline materials applicable, for example, for the flow of ice in glaciers. The answer to the second crucial question was finally found in material science!
Breakthrough! The Great 1960s have started!
1960s: Palaeomagnetic studies, the finding of regular patterns of magnetic anomalies on the sea floor.
1962: Harry Hess suggested that the seafloor was created at the axis of the ridge. In fact, this was a refinement of Molengraaff’s hypothesis.
1965: B. Gordon proposed the quantitative link between solid-state creep and mantle viscosity.
1968: Jason Morgan formulated the basic hypothesis of Plate Tectonics (mosaic of rigid plates in relative motion with respect to one another as a natural consequence of mantle convection).
1968: Isacks and co-workers attributed earthquakes, volcanoes and mountain building to plate boundaries.
1967–1970: Development and broad acceptance of Plate Tectonics. Before this time, continental drift was always opposed by “geophysicists” based on the rigidity of the solid elastic mantle and the ‘absence’ of physical mechanisms allowing horizontal displacements of thousands of kilometres for continents.
Intuition in geodynamics should be – must be – assisted by modeling. Tell us how modeling has assisted in answering some pertinent questions about the earth.
I can give two examples from my personal research experience:
Intuition tells us that rising mantle plumes should always be hotter than the surrounding rocks. However, in subduction zones, chemically buoyant mantle plumes (diapirs) rising from the cold slabs can be colder than the surrounding asthenospheric mantle. This was first hypothesized by Joshi Tamura in 1994. This conceptual idea, however, remained unpopular until such “cold plumes” have been “re-discovered” by Gerya and Yuen (2003) in numerical experiments. Now, this is broadly accepted and a popular concept supported by both numerical models and natural data. Another example of such counter-intuitive cold plumes is the sedimentary diapirs found in the Bushveld layered intrusion. The rise of these structures was triggered by heating of the cold sedimentary floor by the large, hot and dense magma intrusion. Rising, partially molten finger-like sedimentary structures were less dense but colder than the 8 km thick crystallizing “magma sea” surrounding them.
Another example concerns the peculiar orientation of oceanic transform faults that are typically parallel to the spreading direction. Intuition tells us that transform faults are plate fragmentation structures and their orientation is regulated by the state of the stress in extending oceanic plates. Spreading-parallel orientation thus apparently violates a well-known mechanical rule that strike slip faults in extending plates should strike at a significant (>/45o) angle to the extension direction. This “wrong” orientation of oceanic transforms has generated a number of different explanations, for example by the Earth’s sphericity and thermal stresses in cooling plates, which would change stress orientation in the oceanic plates. However, my recent 3D numerical thermo-mechanical modeling of ridgetransform spreading patterns demonstrated to my own surprise that the intuition was actually wrong. These experiments showed that oceanic transform faults are not plate fragmentation structures but rather plate growth structures. This difference in physical origin is to some degree analogous to the difference between a fragment of a thin broken ice plate (ice fragmentation structure) and a snowflake (ice growth structure). Numerical models suggest that the spreading-parallel orientation of transforms is regulated by opening space accommodation within offset ridge segments and not by stress distribution in plates.
Both geology and geophysics are required for understanding geodynamic processes. On global, regional and local scales how do these two disciplines help to understand the different processes taking place on the surface or in the interior of the earth?
We should understand that geoscientists are typically working in a “data-starved” regime and all sources of data are very welcome. Geophysics allows us to “look” inside the interior of the Earth but typically gives only a present-day snapshot. Geology gives geological time records preserved in rocks and structures but these typically formed near the Earth’s surface. If we want to learn about geodynamic processes happening on different scales at all times and at all depths we have to combine both geological and geophysical data. Modeling and intuition can serve as data integration tools.
Tell us about some geodynamically active regions in the world. Also tell us about how geodynamic measurements are made in these areas and how they help in our understanding of the geodynamic picture.
Most active modern geodynamic regions are typically of three sorts: (i) convergent subduction and collision zones, (ii) divergent rifts and mid-ocean ridges, and (iii) magmatically active hot spots. In my opinion, one of the most interesting and rapidly evolving regions of the Earth is located to the southeast of Papua New Guinea. There one finds a “wild” combination of a young, rapidly opening oceanic basin (Woodlark Basin) with a young and active subduction zone that managed to exhume modern ultrahigh-pressure (UHP) metamorphic rocks formed at depths of ~100 km. Most important geodynamic observations comprise high-resolution bathymetric and topographic measurements, GPS measurements, active and passive seismic studies (e.g., seismic tomography and seismic reflection data) and geological-mineralogical rock records (geological and lithological structures, pressure-temperature-time trajectories of rocks, geochronological data).
The present day geodynamic conditions are of fundamental importance, when we study the cause of earthquakes. Tell us how the buildup of stress is conditioned by the geodynamic processes in any area.
Geodynamic processes provide a long-term context for the occurrence of earthquakes. These involve a very slow stage of tectonic stress loading related to long-term tectonic plates movement plus an extremely rapid (runaway) stage of stress release (i.e. the earthquake itself) caused by the development of frictional instabilities inside faults. Very slow geodynamic processes are responsible for both stress distribution and faulting patterns within interacting plates. Understanding how these patterns form and evolve with time, and how and where frictional instabilities develop inside and between plates is one of the major modern geodynamic challenges. Addressing this challenge requires, in particular, significant numerical modeling effort. Modeling is used for testing various long-term geodynamic scenarios and evaluating them in term of possible characteristic spatial-temporal patterns of earthquakes. Robust prediction of earthquakes, however, is beyond the capability of present-day science.
Share This Interview