Materials science is a field that quietly goes about its business, but routinely delivers significant scientific advancements. Somewhere along the way we went from wood, stone, concrete, metal and other basic materials, to metal foams, nano- this and that materials, smart materials, and all sorts of other exotic and useful substances.
Like geophysics, materials science evolved from the combination of several disciplines – mainly chemistry, engineering, and physics. It began in the distant past with ceramics, passed through alchemy, which morphed into the more respected field of metallurgy, after which modern disciplines entered the picture, and eventually things gelled into a distinct field in the 1940’s. Like many modern sciences, advances were driven by World War II and the so-called space race of the 1950’s and 60’s. The Advanced Research Projects Agency in the early 1960’s, a USA government funded program, was a really important factor in the development of materials science and many of its early successes.
Arguably unlike geophysics, materials science is understood and driven by a unifying philosophy, something known as the materials paradigm. What the tetrahedron in Figure 1 captures is how materials scientists go about their business: they study the structure of a material and how it is created, termed processing; this leads to an understanding of its properties; the combination of structure, processing and properties provides a fundamental understanding of its performance; with a grasp of the four blue nodes, they have fully characterised the material and can find applications for it. Figure 1 very clearly shows the distinctness of the four nodes, and their interdependency.
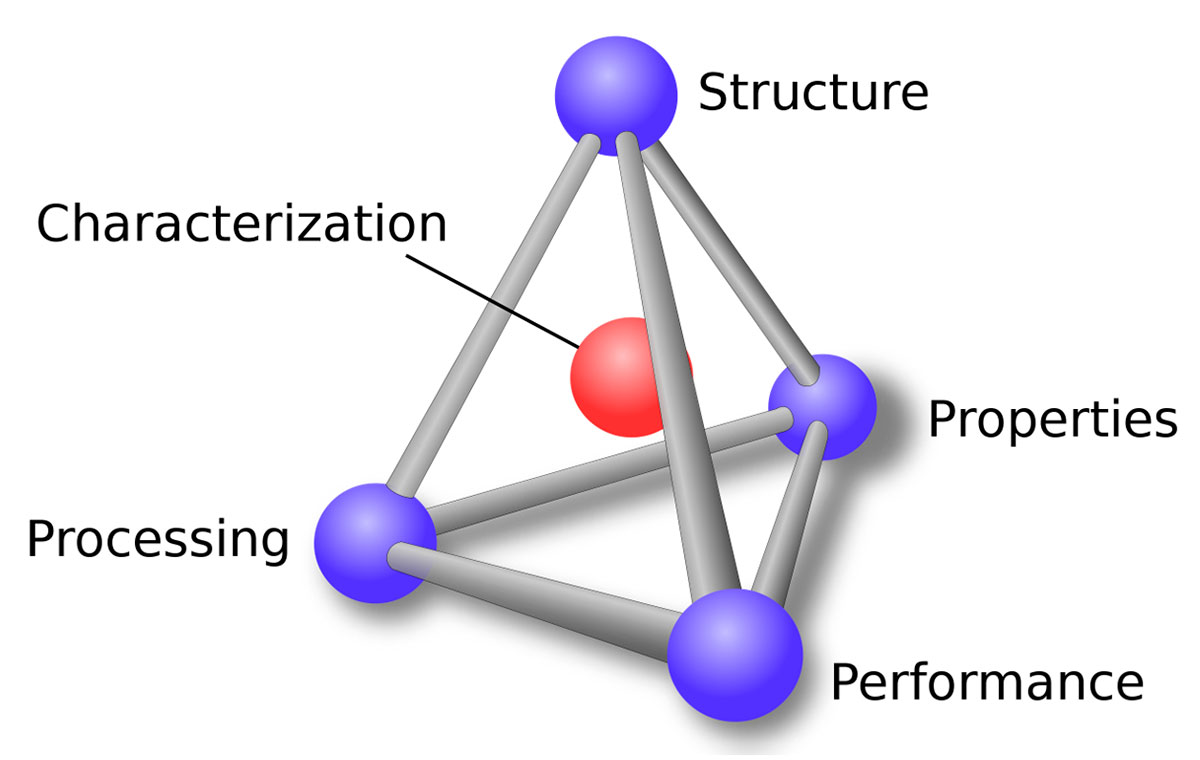
There are many subtleties contained within this paradigm; for example, engineers tend to focus on the interdependencies between performance and processing, while scientists tend to look at the relationships between structure and properties. At any rate, this systematic, bottom-up approach is in contrast to the haphazard, hit-and-miss approach of alchemy, which persisted well into modern times in the development of new materials.
Before taking a look at some interesting new materials, let’s quickly cover the four basic nodes of the materials paradigm tetrahedron.
Structure
There is a heavy emphasis on structure in materials science, probably because structure’s interdependencies with the other three nodes greatly dictate the scope of those nodes. Structure looks at how the components of a material are arranged, at scales that range from the atomic (~10-11m) all the way up to the spans of hundreds, even thousands of metres, involved in larger engineering projects such as bridges and dams.
The influence of structure on properties (which remember, is what we scientists tend to focus on!) is perfectly illustrated with carbon. The differences between diamond and graphite and their properties lie entirely in their different atomic structure. The differences between various carbon materials used in things like tennis racquets, skis, and car parts are determined by their structures at larger scales – is the carbon arranged as fibres, sheets, or blocks? Being a wood worker, I am often thinking about the anisotropic nature of wood, and these are the types of structural considerations materials scientists study to come up with innovative new products. A great example is oriented strand board (OSB), made by bonding previously almost worthless wood flakes into sheets with epoxy; it can then be used to make products like I-beams for construction that are lighter and more rigid in two directions than conventional sawn timbers.
Structure is a huge field and even summarizing it is difficult. There are so many aspects to structure at every scale, and each one is a field in itself. Just think about everything that goes on at the atomic level. Is the bonding metallic, covalent, or ionic? Are there impurities? How are the atoms arranged? Are there different phases at different temperature/pressure regimes? Similar questions can be asked at different physical scales, further complicated by the fact that most materials are a combination of different substances, for example concrete.
Many recent materials science advances have originated from scientists working on structure at the atomic level, especially in the emerging field of nanomaterials. This term refers to materials made of particles with a scale of 100 nM (that’s one hundredth of a millimetre) or less in at least one dimension. These materials often have very different and unexpected properties that can be used in new applications. This has been made possible by technology advances in electron microscopy and other atomic imaging methods, and many new fabrication techniques.
Processing
Processing covers all the steps that affect a material’s structure. Those required to take raw resources to a usable substance are termed primary processes. Mining provides a good example – primary processing starts with the extraction of the raw ore, includes crushing, leaching, refining and all the other steps, and finishes with the production of the basic metal product. Secondary processing covers all the steps that follow which are required to take the basic material to a final product. In the case of metals this would include forging, rolling, milling, alloying, polishing, etc. Note that processing might seem to mainly refer to crude, large scale activities, but often it is these steps that determine a material’s atomic structure. Steel offers a great example of this – it is at the refining stage where things like temperature, rate of cooling, and alloying strategies determine the final steel product’s crystalline structure and thus its properties. I covered this in Science Break: Steel (Kuhn, 2013).
Properties
Again, this is another huge field. For the sake of brevity, I will share Wikipedia’s list of main material property categories (Wikimedia Foundation, Inc., 2021c) – mechanical, chemical, electrical, thermal, optical, magnetic – as well as a reference to their page on this (Wikimedia Foundation, Inc., 2021b) which provides links to the myriad of sub-properties under each category.
Materials scientists mainly focus on the intrinsic properties of a material, that is those that are independent of the amount of material. However, they also look at extrinsic properties that change with the volume of material. They must take into consideration whether a property is a function of any variables, such as pressure, temperature, humidity, etc., and strive to define those functions. Anisotropy is another type of property variable that must be understood. Standardised tests are often used when looking at properties, for example tensile strength.
Performance
This can broadly be described as the suitability of a material for a particular purpose. The criteria used to determine performance could include technical ones, cost, availability, etc. (Materials Science & Engineering Student, 2021) provides a perfect example. When looking at power transmission lines, silver wire has the best conductivity, but it is expensive; copper’s conductivity is almost as good, but it is heavy (and weight greatly impacts transmission line span length). A materials science performance analysis will determine that a steel and aluminum composite wire’s combination of cost, conductivity, tensile strength, and weight provides the best performance.
Material categories
Materials are usually classified into three categories – ceramics, metals, and polymers – and recently a fourth basic class – semiconductors – is often included. The first two we are very familiar with. The term polymer covers a very broad range of materials, usually described as those made up of repetitive arrangements of large molecules, and mainly referring to rubbers and plastics. Semiconductor is also a very broad term, referring to materials with electrical conductivity that falls between conductors and insulators. Recently it has become difficult to place new developments into these four classes, and perhaps they have outlived their usefulness. Researchers at the leading edge of materials science, often using the power and speed of computer modeling and simulation, are investigating the limits of known materials, combining materials in unique ways, mimicking aspects of materials found in nature, and going down all sorts of other avenues, to produce some incredible new materials.
(Wikimedia Foundation, Inc., 2021c) contains a fascinating table of “emerging technologies”, which includes such things as carbon nanotubes, metal foam, programmable materials, and quantum dots. Keep in mind that most innovations produced by materials science are mundane, incremental improvements that we tend not to notice. One example that comes to mind is the gradual improvement in protective hockey gear. Hockey shin pads went from Eaton’s catalogues crammed down oversized socks, to combinations of leather, felt and plastic, to today’s lightweight and almost bulletproof protective gear made from materials such as Kevlar, hydrophobic synthetic leather, nylon, ABS, vinyl nitrile foam, and so on. Over time incremental improvements can produce dramatic changes.
The final section which follows contains a few exciting new materials which could find their way into everyday applications. While it’s easy to sound breathless when describing these materials, materials science has a solid track record of achieving real advances, not just futuristic pie-in-the-sky nonsense. Figure 2 shows the first cell phone from 1973 – over 1 Kg, 10-hour charge time, 30-minute battery life – which had barely any functionality at all, and compare it with what you’re using today. Materials science was behind most of the innovations that made the modern cellphone possible.
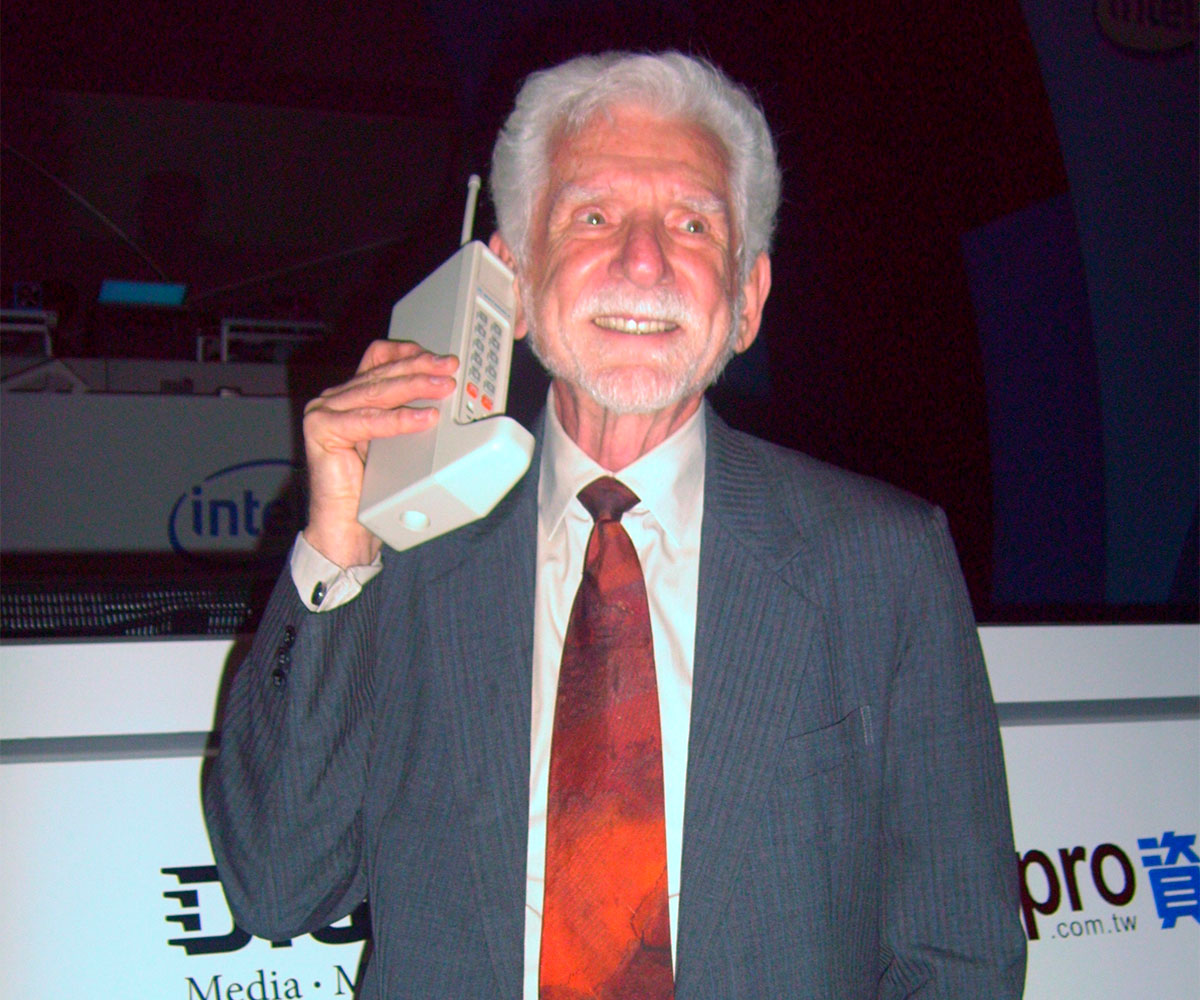
Aerogel
This solid material is created by first mixing a polymer (usually silica) with a solvent to form a gel. Supercritical fluid extraction is then iteratively applied to the gel until all the liquid is removed, leaving behind an extremely light, translucent solid (Figure 3).
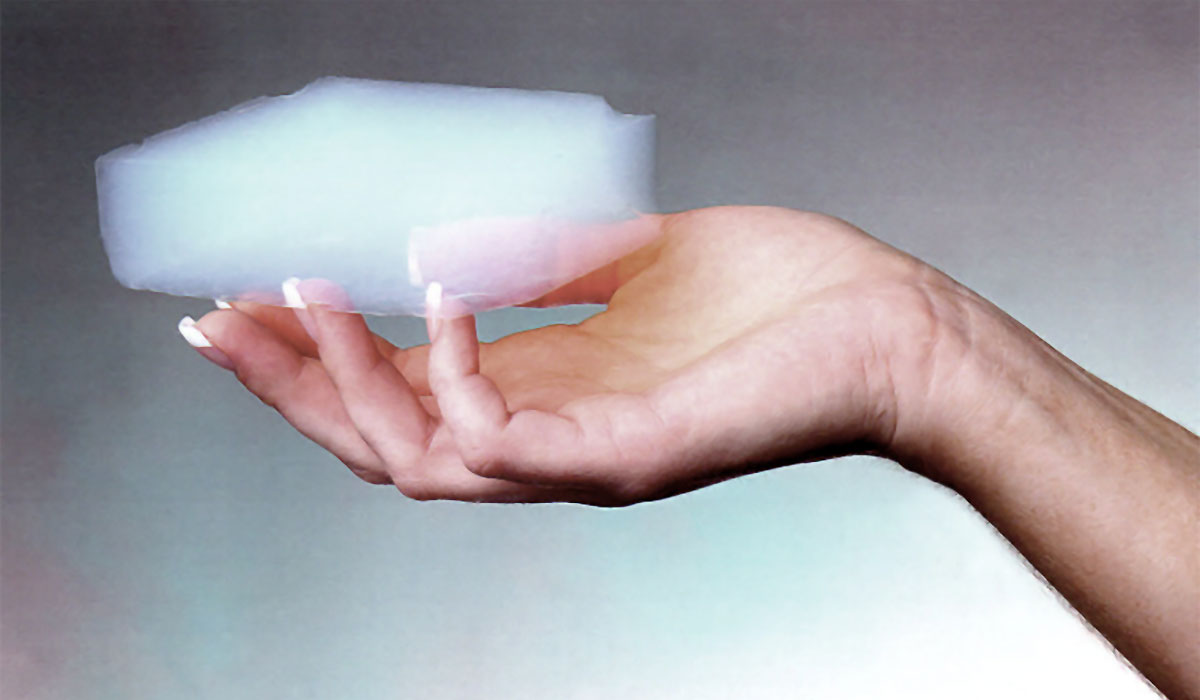
The fluid extraction is done by injecting liquid carbon dioxide into the gel, and then subjecting it to heat and pressure. Once the carbon dioxide reaches its supercritical point, it is no longer a gas or liquid (nor a solid) and it can effuse through the gel, and it is vented out. Aerogels are about 95% porous, and the pores are sized in the nanometer range, much too small to allow air molecules to move through. This makes aerogels extremely good insulators, and this is their most common application. For example, a thin sheet of aerogel can be inserted between two panes of glass to create thin translucent insulating panels. Scientists are actively working on improving the inherent fragility of aerogels.
Carbon nanotubes
To understand the structure of this material requires a short dive into molecular orbital theory. An atomic orbital is a mathematical function that describes the (likely) locations and behaviours of an atom’s wave-like electrons, which can be modeled as regions of variable electron density surrounding the nucleus. Molecular orbitals similarly describe these electron regions within a molecule.
In nanotubes the carbon atoms are held together by the strongest chemical bond known, the sp2 molecular orbital (Figure 4). Without going into too much detail, the s and p indicate the symmetry type of the atom’s orbital, and sp2 describes a situation where an s orbital carbon atom bonds with two p orbital carbon atoms. The result is three equivalent sp2 molecular orbitals oriented towards the corners of a planar triangle, with bond angles of 120º. These molecular triangles are the building blocks for carbon hexagons which are combined to form a mesh sheet one atom thick, which is then wrapped and bonded into a tube (like a very skinny carbon shawarma wrap).
The molecular orbits in diamond molecules, also made of carbon, are sp3, and while these bonds are strong, they are not nearly as strong as sp2. Figure 4 shows the differences between sp2 and sp3 carbon bonding, and how this translates into a totally different macro-structure.
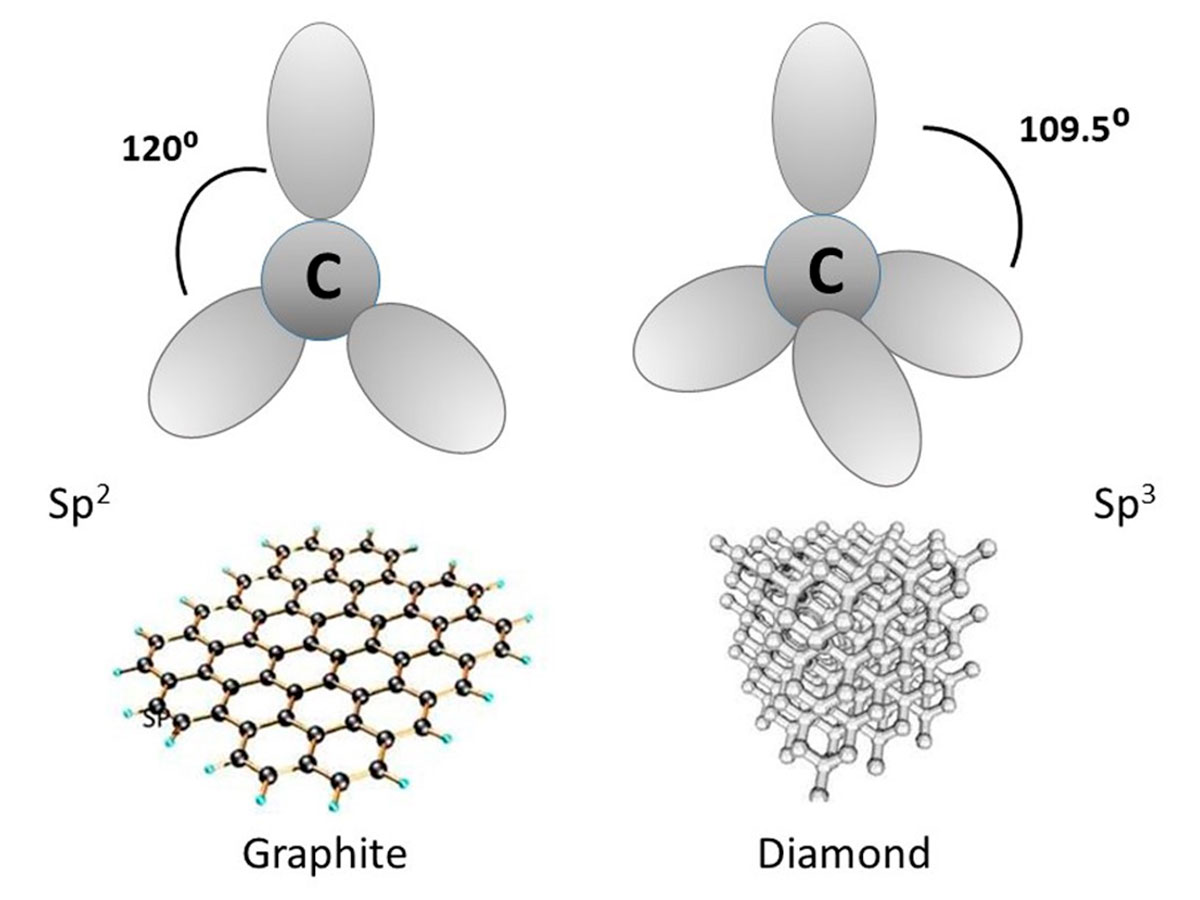
The measured tensile strength of carbon nanotubes is 48,000 kN·m/kg, compared to the 154 kN·m/kg of high-carbon steel. This is a bit misleading. To date nanotube applications have largely used bulk nanotube material made up of unorganised masses of nanotube fragments, and the tensile strength of this material, while high, is nowhere near that of its individual tube constituents. Figure 5 is an image of aligned carbon nanotubes, and this arrangement would have much higher tensile strength. Regardless, nanotubes have already found their way into our everyday objects, including sports equipment, wind turbine blades, as well as obscure applications such as providing the framework for synthetically grown bone tissue. Working within their materials tetrahedron, scientists and engineers are creating new materials from nanotubes (which are not limited to carbon) all the time. For example, nanotube fibres already have been created, and clothing made from these materials is probably not far off, perhaps with some exotic optional properties, such as electrical heating and lighting.
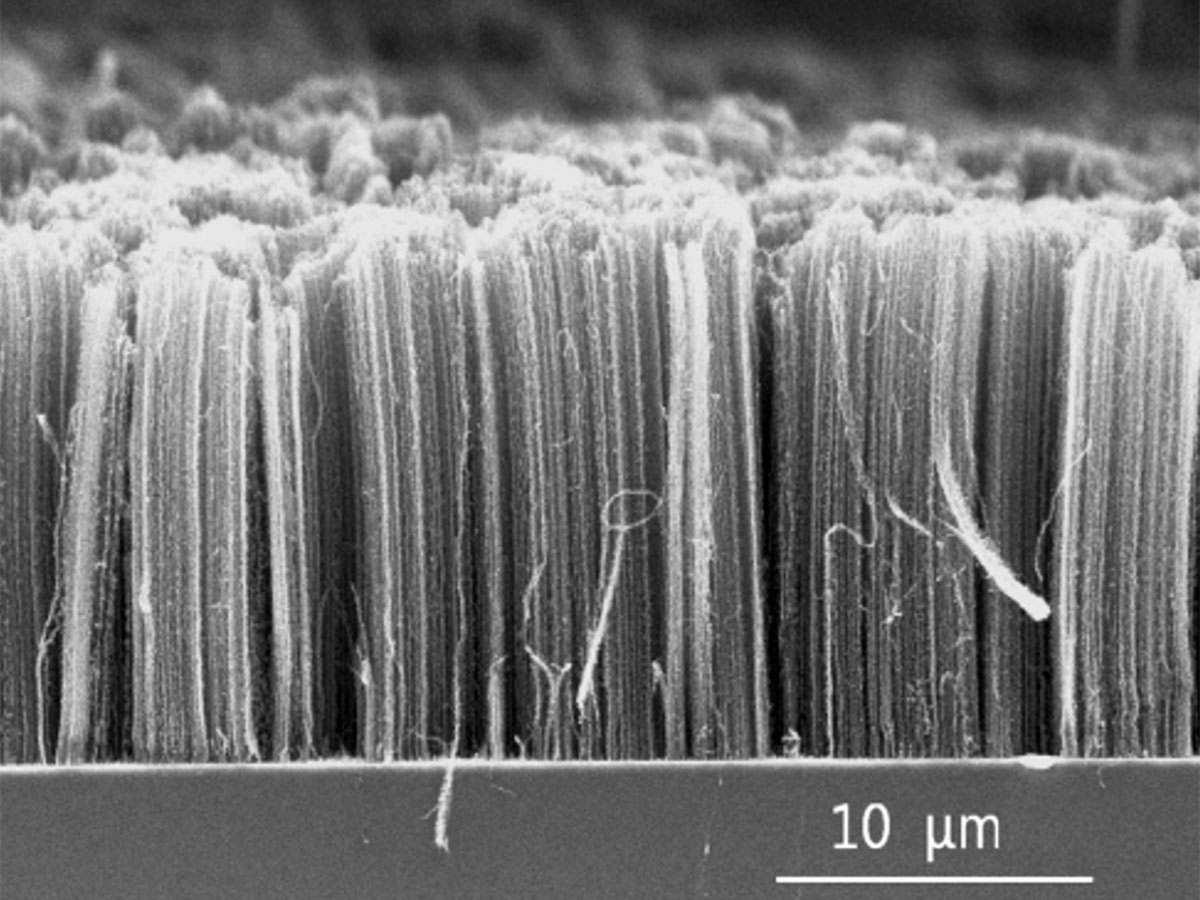
Bulk diamond
I’m particularly intrigued by this material. A process called chemical vapour deposition used to create artificial diamonds is now able to create thick, continuous sheets of diamond, taking it from the relatively small crystals we know as diamonds, towards possible uses as a bulk material, perhaps replacing materials such as metal, wood and concrete. Diamond is very strong and light, can be transparent, has a high melting point, and from an economic perspective, is made from an extremely abundant element. It should be fairly easy to introduce impurities during its creation to give it various properties, just as we do with metals. I think the use of bulk diamond in buildings and machines is feasible and likely, if the materials science behind synthetic diamonds continues to progress, and is perhaps combined with other emerging technologies such as 3D printing. I think it would be funny if diamonds went from being among the most overpriced and useless baubles (I’m ignoring their industrial uses of course) to a very widely used low-cost and high-performance material.
Conclusion
The Internet is a perfect place to find examples of new materials, and there is no need to replicate that here. Beyond the wow factor of new materials science innovations, I am most intrigued by the philosophy underpinning the field. We tend to view the process of invention as one that combines genius and luck, and most certainly that does still occur, but the achievements of materials science are best viewed as a testament to rigour, rationality and basic scientific principles.
Share This Column