The sky is falling! The sky is falling! Well, not usually, but Chicken Little is occasionally right. There are times when stones and ice do fall from the sky. And if they’re big enough, they’ll excavate major cavities on impact. After erosion, infilling, and burial, these impact craters can become significant hydrocarbon reservoirs and even have their own source rock. Impact features on Earth’s surface have traditionally been identified by their topographic character - circular hills - like a large bowl with an upturned rim and sometimes uplifted centre. Their identification is fairly straightforward on the surface and can be confirmed by detailed petrographic analysis of rock samples. Many craters have been thus found. But we’re also interested in the subsurface ones as they might have hydrocarbon accumulations. So, we have to resort to methods such as seismic, gravity, and magnetics to find these buried structures. Impact craters are often highly eroded before burial, but their unique geophysical characteristics can aid in recognition. We have examined a number of geophysical datasets collected within the Western Canadian Sedimentary Basin (WCSB) and other parts of the world over circular subsurface features. These structures have many characteristics diagnostic of meteorite impact craters such as raised rims, annular synforms, terraced regions, inferred breccia infill, and large-displacement faults.
Impact Craters: What are they and how many are there?
Characterized by their size and morphology, impact craters are classified as either simple or complex. A ‘simple crater’ (Figure 1) is distinguished by a bowl-shaped cavity partially filled with a breccia lens. Simple impact craters also tend to have a well-defined rim and are regular in shape (when formed by a projectile moving in excess of a few kilometers per second). The rim-to-floor depth is roughly one-fifth of the rim-to-rim diameter, and the rim height is about 4 percent of the total diameter (Melosh, 1989). The maximum diameter of such a crater is largely dependent on the surface gravity of the impacted body with additional influence from the strength of the impacted surface. On the Earth, this limit is approximately 4-km in igneous rock and 2-km in sedimentary rock. Beyond this limit, the morphology of an impact crater changes dramatically due to gravity flattening the crater. The formation of a central uplift and slump blocks arranged within an annular synform characterizes a ‘complex crater’ (Figure 2).
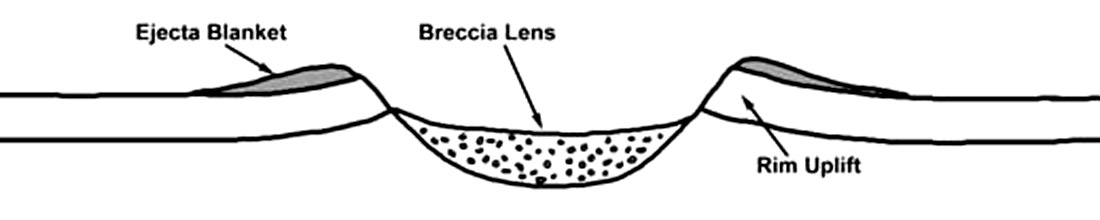
Complex craters are much shallower for their diameter than simple craters and appear to represent collapsed simple craters. The material making up the central peak is composed of rocks that originated below the crater floor. The total amount of stratigraphic uplift is roughly 8 percent of the crater’s final diameter (Melosh, 1989). In both types of craters, an ejecta blanket is present. Often, overturned stratigraphic features are noticed around the rim of simple craters or on top of the slumped blocks in a complex crater.
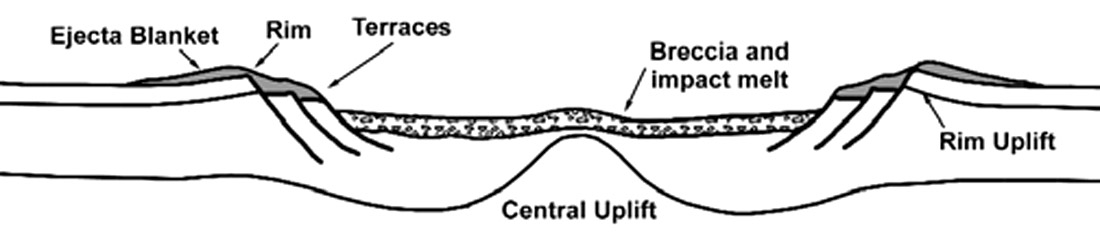
As crater size increases, the central peak of a complex crater changes to a concentric ring of peaks. The peak ring diameter doesn’t exceed approximately half the rim-to-rim diameter. The peak to peak-ring transition diameter scales in the same manner as the simple to complex transition diameter, inversely with the gravitational acceleration.
Both simple and complex craters exhibit a raised rim structure that is due to both ejecta deposits and structural uplift of the underlying preimpact surface. This uplift is greatest at the rim crest and disappears at approximately 1.3 to 1.7 crater radii from the center (Melosh, 1989). As the crater grows, strong radial forces tend to squish the rock units upwards.
Large amounts of seismic data have been acquired within the WCSB in the quest for hydrocarbons. Some of these data show enigmatic circular features that are not easily explained as reefs, diatremes, or dissolution features (Sawatzky, 1976; Isaac and Stewart, 1993). Nevertheless, these features are of interest to resource companies as they often prove to be productive in terms of hydrocarbons.
Diameter (km) |
Impact Frequency (per 600 MY, Earth) | Impact Frequency (per 600 MY, Alberta) | Impact Frequency (per 600 MY, WCSB) |
---|---|---|---|
Table 1. The frequency of impacts over the past 600 MY distributed over the Earth, within Alberta, and within the WCSB. (after French, 1998) | |||
>1 | 384000 | 494 | 988 |
>1.8 | 138000 | 177 | 354 |
>3.1 | 49800 | 64 | 128 |
>5 | 21000 | 27 | 54 |
>7.2 | 10800 | 14 | 28 |
>10 | 6000 | 8 | 16 |
>12.2 | 4260 | 5 | 10 |
>20 | 1740 | 2 | 4 |
>31 | 840 | 1 | 2 |
>50 | 132 | 0.2 | 0.4 |
>100 | 24 | 0.03 | 0.06 |
Over the past 30 years, much work has been done to determine the terrestrial cratering rate during the past several hundred million years. These studies are based on cratering statistics on fractions of the Earth’s surface where all the large craters are believed found and the age of the surface may be estimated (Grieve, 1998). Examining a large sedimentary basin, such as the WCSB, we can estimate the total of number of impacts that resulted in craters larger than any given size during its history (approx. 600 MY for the WCSB). For Alberta, we expect that there have been approximately 500 impacts that resulted in craters larger than about 1 km in diameter (Table 1). Although erosion has left large stratigraphic gaps in the geologic column, a crater larger than 1 km in diameter will affect strata to depths greater than 500 m below the original surface. As a result, many of the craters larger than 1 km in diameter should survive most periods of erosion and should be visible on seismic data. Figure 3 shows a random distribution of craters based on the expected terrestrial cratering rate versus the known possible and confirmed impact structures in Alberta. Since the major sedimentary basins around the world are generally well imaged by seismic and other means, there are likely many impact structures ‘hidden’ in these datasets simply awaiting discovery. For example, in the province of Alberta most of the WCSB has been sampled by 2-D seismic reflection surveys at a line spacing of less than 1 km, implying that most impact structures greater than 1 km diameter will have been crossed by at least one survey line. As structure in the basin is sought by explorationists, we assume that such structures, once detected, will often be further explored. It remains a source of speculation why more craters have not been identified. It may be that structures located in the deeper portions of the basin have not been detected, but the recognition of the James River structure at ~4.5 km depth (Isaac and Stewart, 1993) indicates that at least some deeply located candidates are detectable. It is also likely that a significant number of structures have been observed but misidentified as being of non-impact origin.
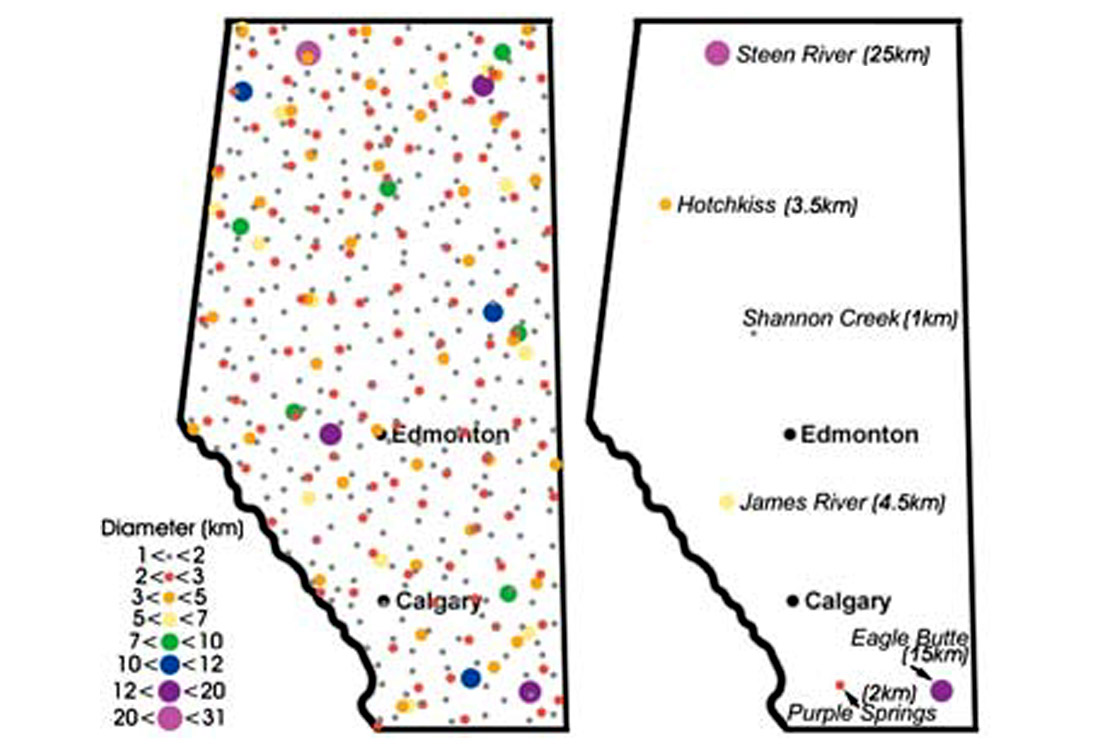
By using the large amounts of seismic data collected in the sedimentary basins around the world, we expect to find dozens of new examples of buried impact features. We also expect that the use of seismic interpretation methods will help us improve our understanding of the formation mechanics and structure of impact craters.
Why study impact craters?
Of the more than 150 known impact craters on the Earth (Figure 4), approximately 25% have been associated with economic deposits at one time or another. Of these, 17 are being actively exploited (Grieve and Masaytis, 1994). In some cases, the deposits are world class such as the Cu-Ni deposits at Sudbury, Ontario. Impact craters also make excellent hydrocarbon reservoirs as evidenced in table 2. Ames Hole, for example has a number of wells in production with several producing more than 500 BOPD from the crater rim and floor in 1995 (Carpenter and Carlson, 1997). In Alberta, the Steen River structure (Figure 5) has been explored for the past 30 years but, due to a lack of infrastructure, its hydrocarbon potential has only been realized recently.
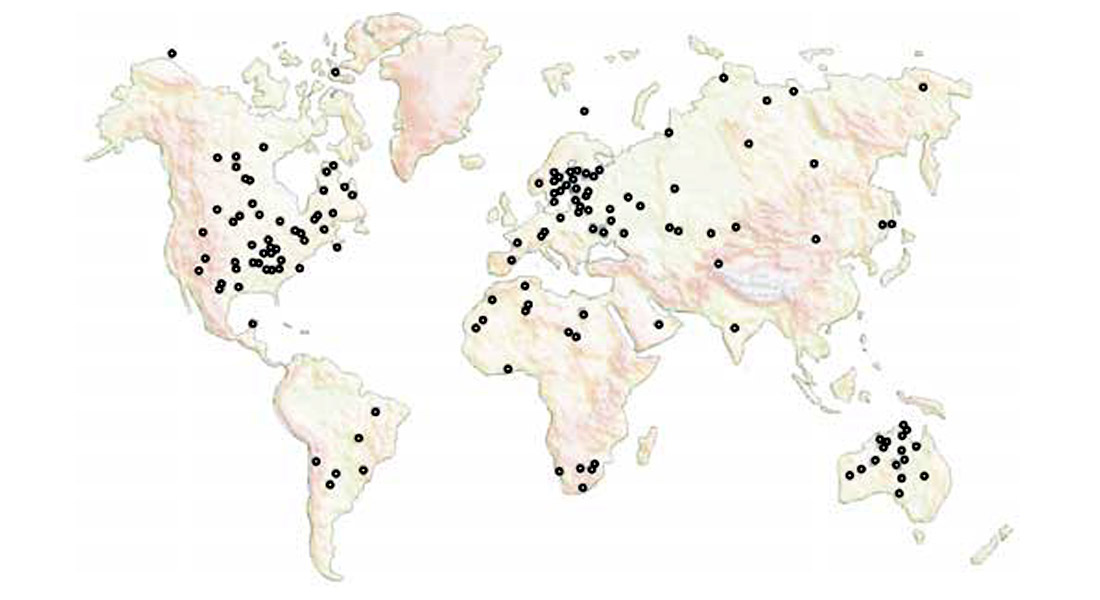
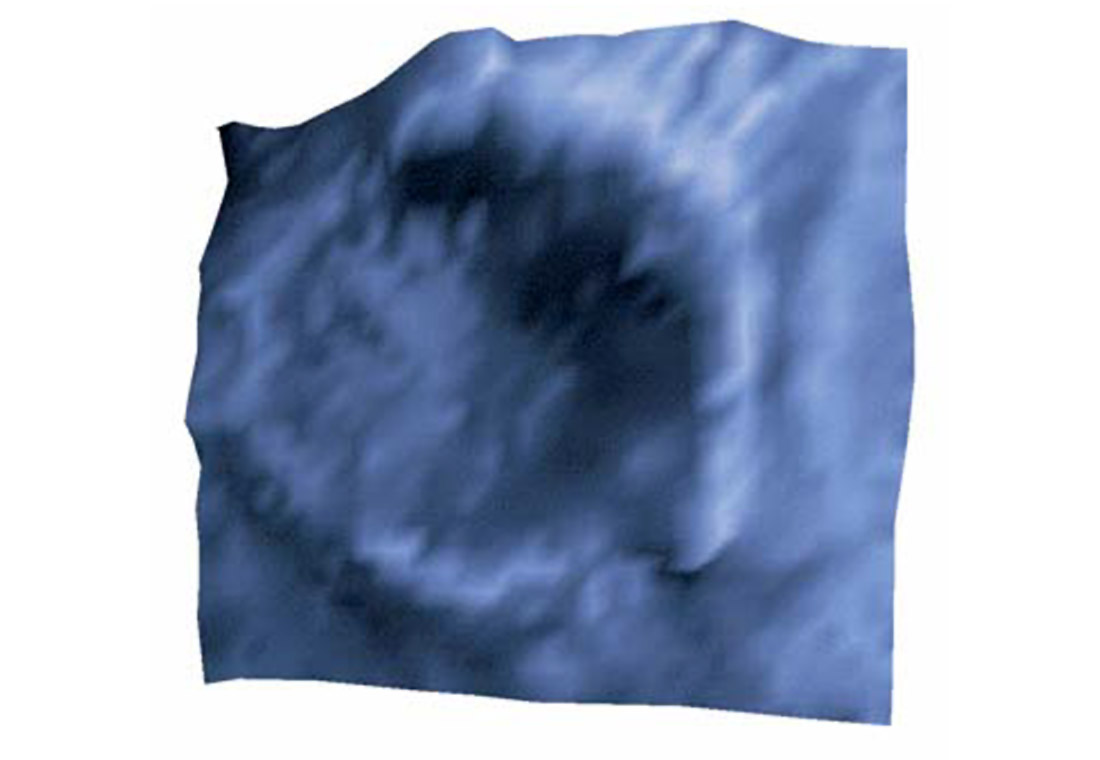
Finding a crater: Seismic methods
Early searches for terrestrial impact craters were done by examining topographic features and drainage patterns for evidence of circular structures. However, many processes in nature exist that may produce round features that can be confused with impact craters. Diatreme intrusions, salt and shale plugs, volcanoes, igneous intrusions, and dissolution features can all result in circular structures. Their subsurface appearance, as revealed by seismic imaging, can help determine the process responsible for their creation.
Impact Feature | Diameter (km) | Age (MY) | Hydrocarbon Accumulation |
---|---|---|---|
Table 2. Hydrocarbon production associated with several impact craters in North America (adapted from Isaac and Stewart, 1993). | |||
Ames Hole, Oklahoma | 8 | 450 | 2x106m3 (18MMbbl) oil and 4x108m3 (14 BCF) gas in estimated reserves from crater rim and floor |
Avak, Alaska | 12 | 3-100 | 109m3 (37BCF) gas reserves in slump block traps |
Calvin, Michigan | 72x106m3 (600MMbbl) oil | ||
Newporte, North Dakota | 3.2 | 500 | Oil shows and some production from raised rim |
Red Wing Creek, North Dakota | 10 | 200 | 6.4x106m3 to 11x106m3 (40-70MMbbl) in recoverable reserves from the central uplift |
Steen River, Alberta | 25 | 97.5 | Oil (~1000 BOPD) and gas production (~35 MMCF/d) from rim structure with 2x109m3 (72 BCF) gas reserves |
Tookoonooka, Australia | 55 | Potential for stratigraphic traps | |
Viewfield, Saskatchewan | 2.4 | 200 | 3.2x106m3 (27MMbbl) reserves from raised rim |
Due to the economic interest in structural plays within the WCSB, much of the basin has been well imaged by seismic means. Most of this data has been thoroughly interpreted with several seismic examples of possible impact structures noted in the literature (Sawatzky, 1972; Isaac and Stewart, 1993; Westbroek, 1998; Mazur, 1999). These range from the ~1 km Shannon Creek structure to the 25 km diameter Steen River structure in NW Alberta.
By providing an image of contrasting lithologies in the subsurface, the seismic method can allow one to identify characteristics diagnostic of meteorite impact features. These features may include: down-thrown slump blocks, uplifted rocks at both the rim and central uplift, breccia infill, melt sheets, and zones of structural pinching. Figure 6 shows a preliminary interpretation of the ~500 MY old Muskingum structure in central Ohio. This feature is about 1.3 km across and is believed to be an eroded impact crater. Diagnostic characteristics include a bowl-shaped cavity whose dimensions are consistent with established crater scaling relations, central mounding, possible brecciated regions, faulting, and lack of evidence for a diatreme pipe. Although this simple structure is relatively uneroded, a great number of similarly-sized craters are eroded beyond the limits of detection. As a result we find that a large proportion of seismic examples of suspected impact craters are over the larger complex structures.
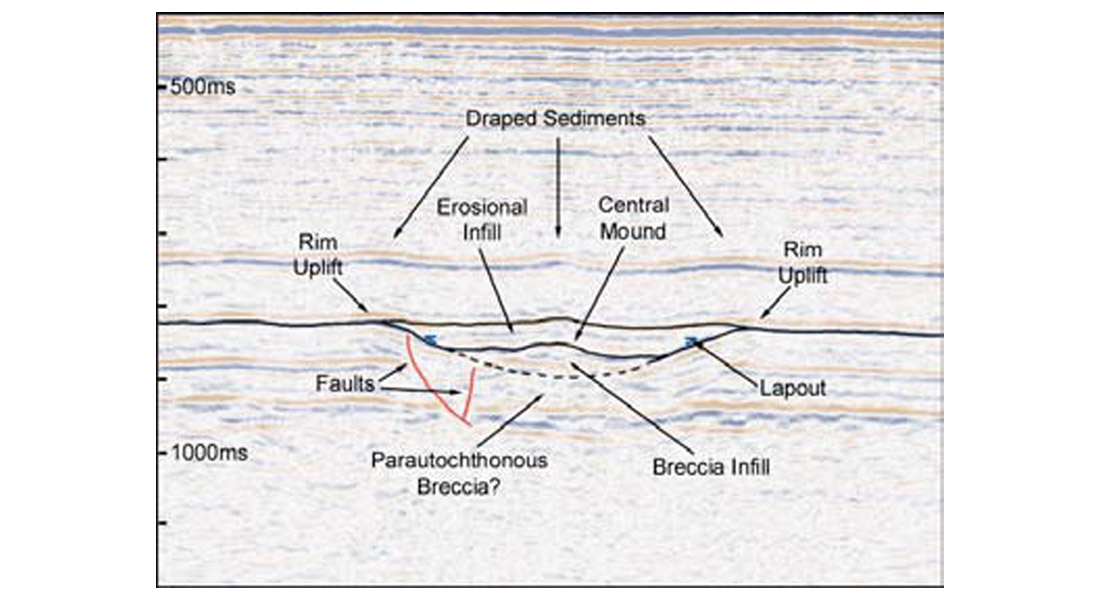
Figure 7 shows the seismic appearance of a possible 120-330 MY old complex impact structure in NW Alberta. The Hotchkiss structure is approximately 3.5 km in diameter; a central uplift, slump blocks, and a possible breccia infill may be interpreted. Zones of velocity pull-up appear to be well correlated with the trough on either side of the uplift. Lateral velocity variations across the section thus imply an infill material that is different than regional stratigraphy. Similar to the Hotchkiss structure, the White Valley structure (Figure 8) is a relatively small (~6 km) structure showing many of the characteristics of a complex impact feature. Apparent on the seismic data is evidence for a central uplift, slump blocks, and structural pinching. Although the seismic data from each of these structures provides evidence for impact generated structure, the final determination of their origins will come from studies of core/chip material for shock metamorphism features.
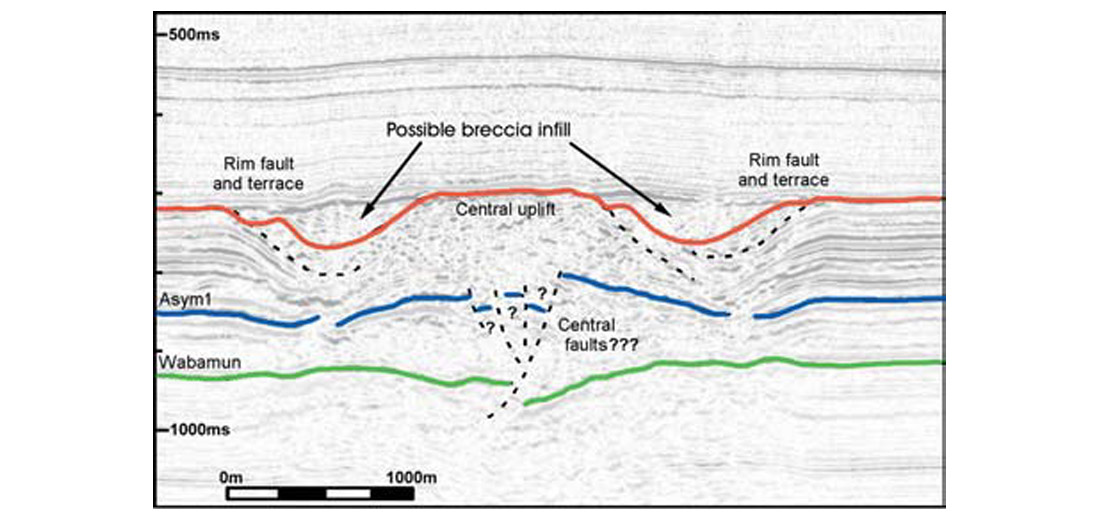
3-D seismic: The best method?
With the increasing use of 3-D seismic as an exploration method, the method’s potential to the study of subsurface craters are noteworthy. As with any structural play, 3-D seismic will help to better define the extent of interesting structures and will aid in determining whether the necessary closure for structural traps is present.
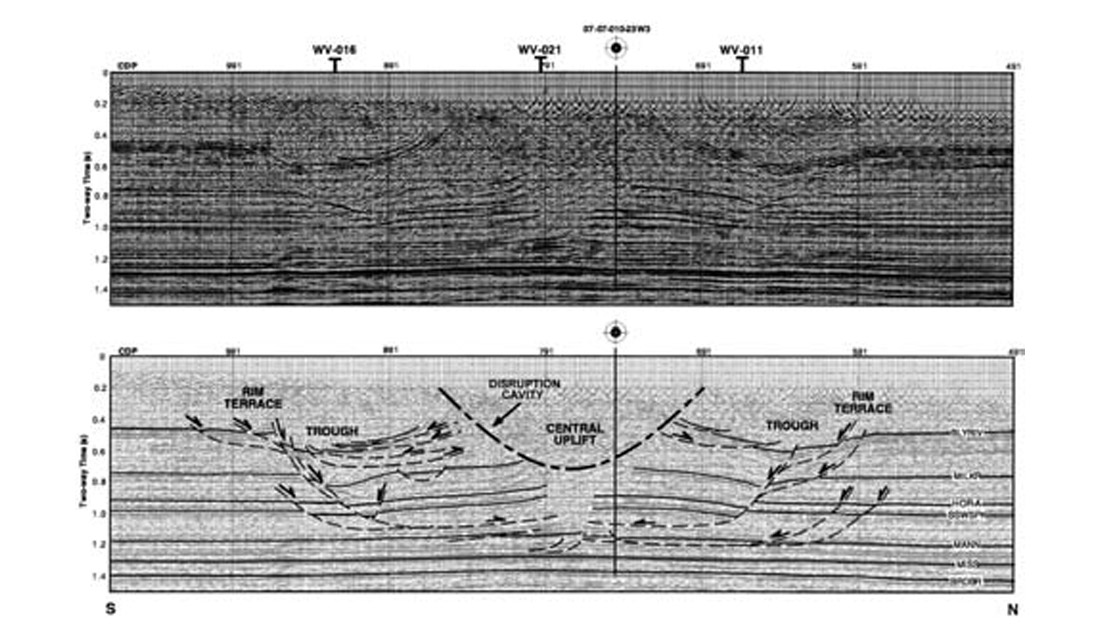
Located in south-central Alberta, the James River structure (Figure 9) is a possible complex impact 5 km in diameter buried beneath nearly five kilometers of sediments. Although the disturbed Cambrian rocks are not of economic interest, topographic features related to the feature appear to have aided in the formation of reefs found at shallower depths.
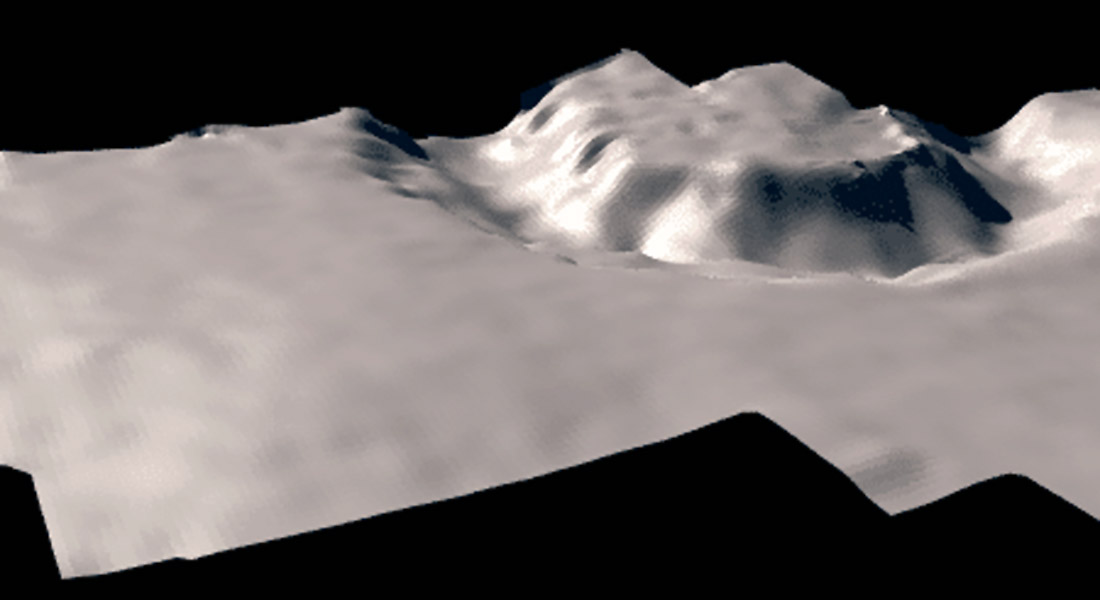
Also imaged by 3- D methods is the Puffin structure (Figure 10) in the Timor Sea on the northern Australian continental shelf. Its initial appearance is that of a nearly circular feature with a flat floor and rim uplift. Is this an example of a well-preserved simple impact structure? It is difficult to say, but the carbonate environment and the presence of faulting imply that this feature may actually be the result of dissolution. This remains an interpretation problem subject to further constraints.
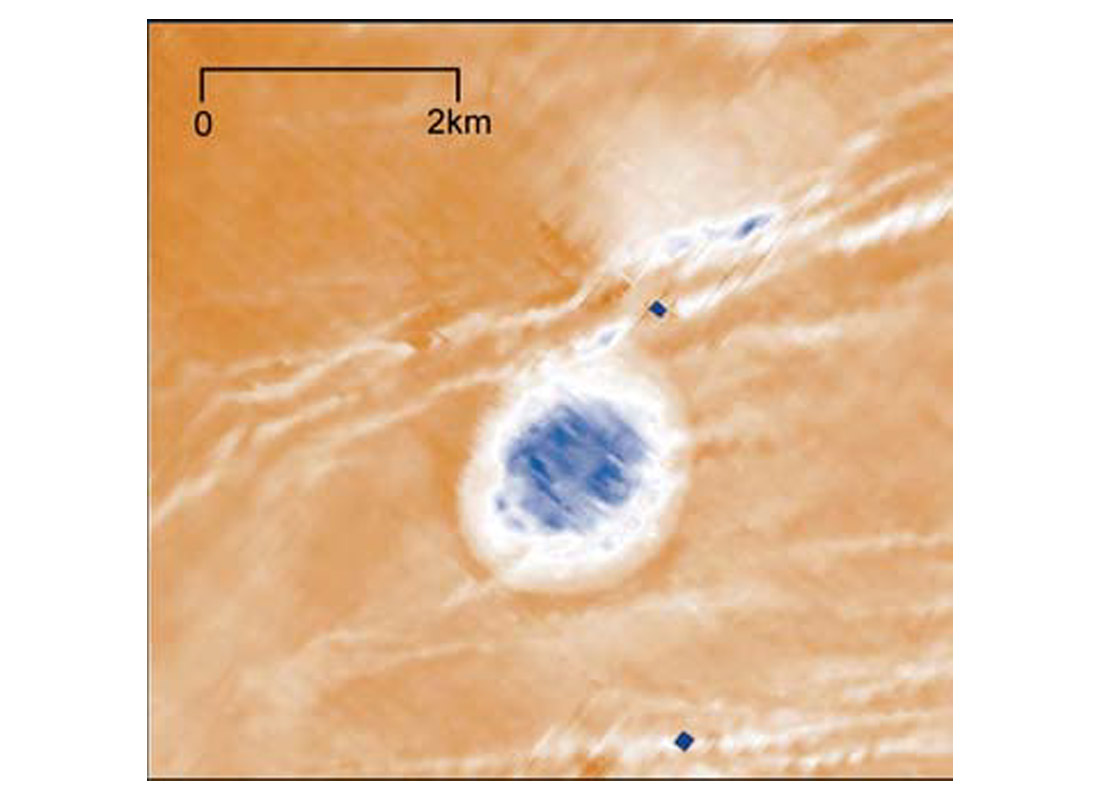
Where are the hydrocarbons?
As with other types of potential hydrocarbon reservoirs we look for indications of a source, structure, and signs of a seal. Due to the highly distorted structures of impact craters several many different kinds of potential reservoirs are created. The breccia infill, the rim uplift, and the central uplift all provide the structure necessary to collect hydrocarbons, and production currently occurs from these components. Subsequent sedimentation in craters can provide both a source rock for hydrocarbons and the seal for crater structural elements. Other potential structural traps are those formed reef growth on the rim and sediment draping over the buried and subsiding crater.
Summary
It is well established that extraterrestrial objects large enough to form impact craters larger than 1 km in diameter occasionally bombard the Earth. In fact, when one considers the currently accepted terrestrial cratering rate, it is found that nearly 500 such impacts have occurred within Alberta over the last 600 MY. Although many of these features have been erased by erosion, several hundred should still be visible in the sedimentary record. Due to the dense seismic coverage in the WCSB we expect the interpretation of possible impact structures from this area to play a useful role in future studies of the terrestrial cratering record. Also, the economic importance of impact craters in the WCSB is substantial. Nearly 25% of all known impact features currently have some economic potential. When one then considers the expected number of buried craters, this makes impact-related features possibly one of the most under appreciated structural plays in the WCSB.
Acknowledgements
The authors would like to thank all of those involved with this project. Several CREWES project sponsors (Gulf Canada, Husky Oil, Shell Canada Ltd.) and others (BHP Petroleum Pty., CGAS Exploration Ltd., Pulse) have been instrumental by providing much of the seismic data that we are currently working on. As always, we are interested in learning of other seismic datasets showing some of the characteristics discussed here.
Join the Conversation
Interested in starting, or contributing to a conversation about an article or issue of the RECORDER? Join our CSEG LinkedIn Group.
Share This Article