Introduction
It is well known that the production of conventional light from the Western Canada Sedimentary Basin is declining rapidly. Heavy oils, here taken in the broad sense to include both heavy oil and bitumen, are replacing much of this lost production and their combined production already exceeds that of conventional oils.
There are numerous Heavy Oil Plays in Western Canada; an updated and detailed report has recently been published by the National Energy Board of Canada outlining this resource (NEB 2001b) which suggests there is nearly 8 X 109 m3 of in-place heavy oils of which upwards of 20% (~1.5 X 109 m3) is recoverable using current technology. There are important Carboniferous and Jurassic plays, but about 70% of the heavy oil is in the Cretaceous age deposits, with the Lloydminster area of Saskatchewan and Alberta having the largest part of the resource.
Although there are some minor oil sand deposits on Melville Island, the vast bulk of Canada’s bitumen lies within sands and carbonates in three areas defined as the Athabasca, the Cold Lake, and the Peace River oil sands. The total area that encloses these deposits is the size of New Brunswick (~60,000 km2). The National Energy Board estimates that there is potentially 400 X 109 m3 bitumen in place of which ~50 X 109 m3 is ultimately recoverable. This is comparable to Saudi Arabia’s conventional reserves of 43 X 109 m3 and is many times larger than Alberta’s conventional reserves of 0.7 X 109 m3 (DOE 2000).
Despite these large reserves, however, heavy oils are highly viscous and their extraction is more complicated; this will have large implications for the geophysical community as some emphasis will shift away from exploration to development and monitoring issues. This report attempts to provide some background information on the physical properties and the methods of production of heavy oils in the Western Canada Sedimentary Basin. Canadian Geoscientists have made early inroads with the use of geophysics in monitoring of such reservoirs and this work is reviewed. Recent research directions are also summarized.
Heavy Oils
What characterizes heavy oils
Two physical properties best define the heavy oils – their density and their viscosity. Both are related to their chemical composition; natural oils are composed of numerous different organic compounds. Heavy oils, however, differ primarily from lighter, less viscous oils in that they include larger proportions of large molecular weight organic compounds including complex asphaltene molecules. This is best seen in the overall elemental composition in that the heavy oils are deficient in hydrogen (Table 1) relative to conventional light oils; generally a greater hydrogen:carbon ratio equates to a higher energy content. Upgrading of heavy oils and bitumens to make synthetic oil requires that hydrogen be added. Heavy oils further contain higher levels of sulphur that must be removed during upgrading. Many of the heavy oils in the Western Canada Sedimentary migrated updip from the west and during this period of migration had to have lower viscosities. The chemistry of these original oils was in many cases changed by bacteria removing the lighter portions and leaving only the heavier organic residues.
TABLE 1
The low hydrogen:carbon ratio means that the heavy oils are composed of larger organic molecules and nitrogen containing organics such as carbazole (C12H9N). These differ from lighter organics by having substantially higher melting temperatures, densities, and viscosities. Indeed, it is density that is the characteristic that most directly separates the heavy from the light oils. In Canada, oils with densities greater than 900 kg/m3 are classified as ‘heavy’ (Figure 1) whereas under the API designation scheme ‘heavy’ refers to API specific gravities of between 10 and 22 with ‘bitumen’ meaning those oils with densities greater than that of water (1000 kg/m3).
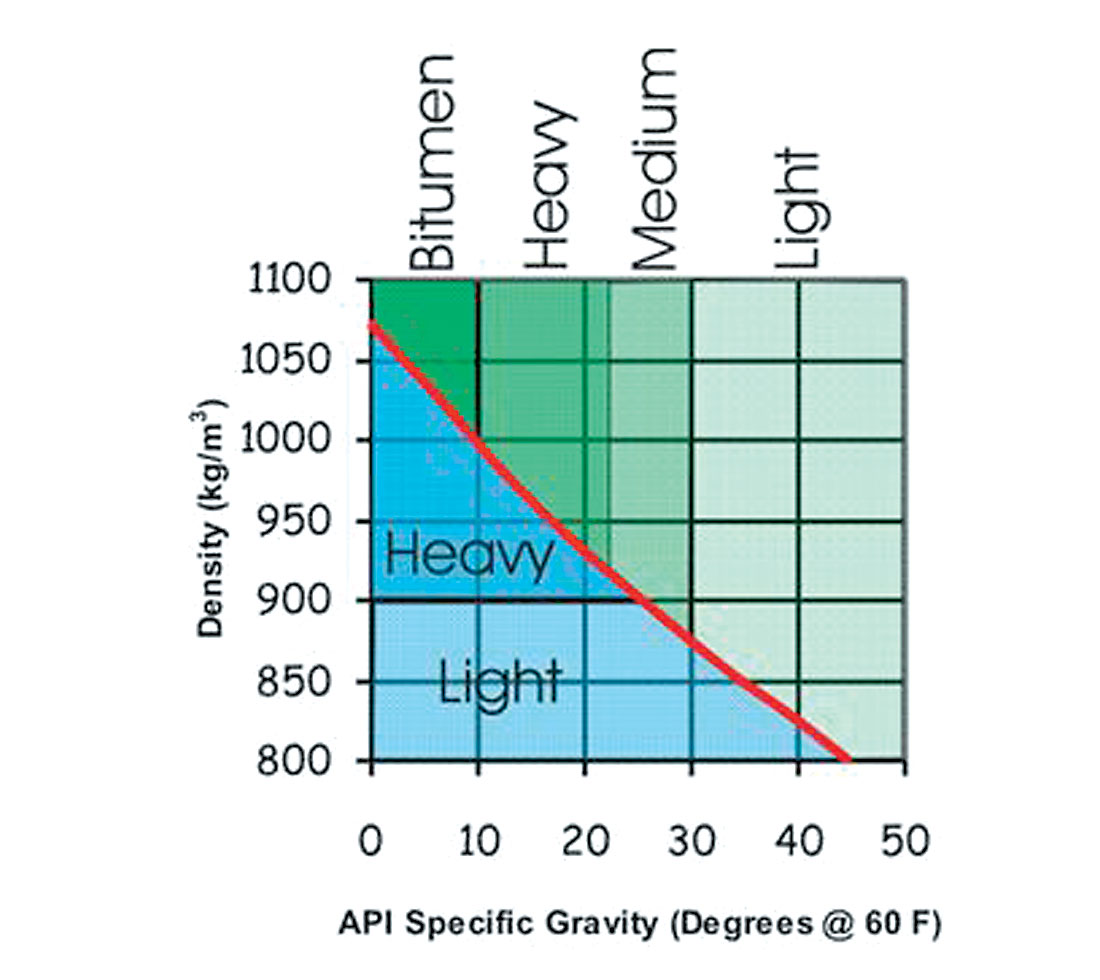
Viscosity is the second critical property of heavy oils; this is very important in that their high viscosities limit their productivity. The viscosities of conventional light oils are only slightly greater than water and hence are able to flow in a reservoir with relative ease given sufficient permeability. The viscosity of heavy oils already exceeds this by three orders of magnitude and while in some reservoirs flow is possible, it is obviously much more restricted. In the bituminous reservoirs, however, the viscosity is again increased by up to two more orders of magnitude; these oils are for practical purposes immobile under typical reservoir temperatures.
TABLE 2
Special measures need to be taken in order to recover these oils. Many of these measures exploit the fact that the viscosity declines dramatically with temperature (Figure 2). The viscosity of a typical near surface Athabasca bitumen, for example, at a virgin reservoir temperature of about 20ºC declines by up to six orders of magnitude (~107 to 101) upon heating to temperatures near 225ºC. In the absence of other factors, this gives the heavy oil a viscosity not that much different from conventional light oils. In addition to heating, the viscosity can also be lowered by injecting various solvents such as CO2, or lighter hydrocarbons such as propane, although the use of such solvents is still experimental.
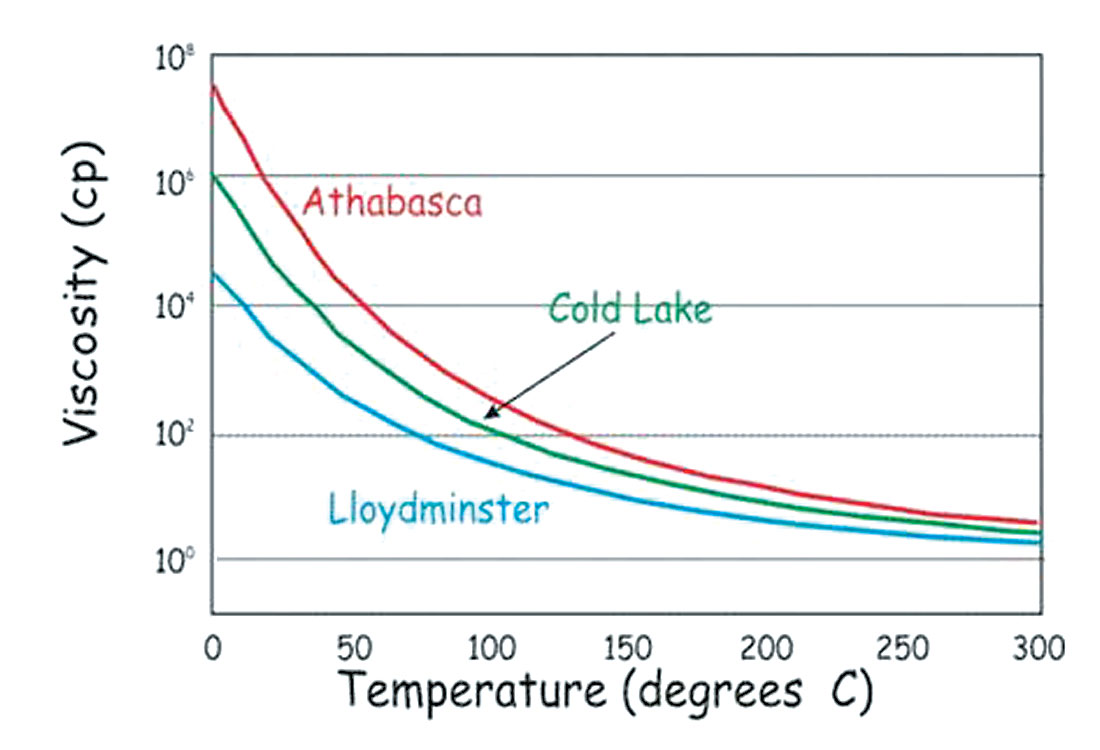
Production Strategies
As noted the viscosity of these oils is high, and the material will not flow naturally in many cases. A number of different schemes are used to lower this viscosity in order that the oil becomes mobile and can be produced. In the early days of nuclear energy it was even suggested that low yield nuclear explosions might be able to provide the heat to mobilize the oil (Alberta Technical Committee, 1959) but thankfully this option was not explored further. While most of the bitumen production today comes from surface open pit mining operations, more than 80% of the recoverable bitumen is at depths greater than 75m, at which point truck and shovel mining is no longer considered economic. To overcome these difficulties, a number of in situ recovery schemes have been or are in development. Some of the more important production methods include:
Cold Production: In cold production heavy oil is obtained by allowing a sand-oil mixture to be pumped out using special progressive cavity pumps. Allowing a small amount of loose sand to be produced appears to increase production rates nearly 10-fold. This increase is due to an improved overall effective ‘surface’ area of the wellbore. The reason for the greater delivery is still being actively debated but one of the more intriguing explanations suggests the sand and oil is removed via long, narrow ‘wormholes’ that give the borehole a very large effective surface area. Such features have been created in the laboratory by the Alberta Research Council. However, cold production recovers only a small fraction of the reservoir’s oil, perhaps about 15%.
Cyclic Steam Stimulation: In Cyclic Steam Stimulation, hot water and steam at temperatures near 300°C care injected to an oil sand reservoir. The hot water is sufficiently pressurized in the hopes that the reservoir materials will be fractured to allow for better fluid communication. One cycle typically consists of periods of high pressure steam injection followed by a shut in ‘soaking’ period to allow for transfer of heat to the reservoir, followed finally by a period of production. Once production rates decline a second cycle begins with reinjection of steam. This method is operating on over 3000 wells in the Cold Lake deposits.
Steam Assisted Gravity Drainage: This technique relies on a slow, steady pressurization of the reservoir with steam from one horizontal wellbore and heated oil is produced via a second parallel wellbore drilled immediately beneath the injector. In concept, steam occupies the pore space immediately above the injector and heated oil drains around the outside of the steam chamber driven by gravity flow down to the producing wellbore. Steam assisted gravity drainage, or SAGD, relies on a number of technical innovations including highly steerable horizontal drilling. This method is now being applied at a commercial level at a number of different sites in Saskatchewan and Alberta. A variation on this idea is the VAPEX concept in which an injected solvent is instead used to lower the oil viscosity. This technique would apply to thin reservoirs where conductive heat losses make steam injection uneconomic.
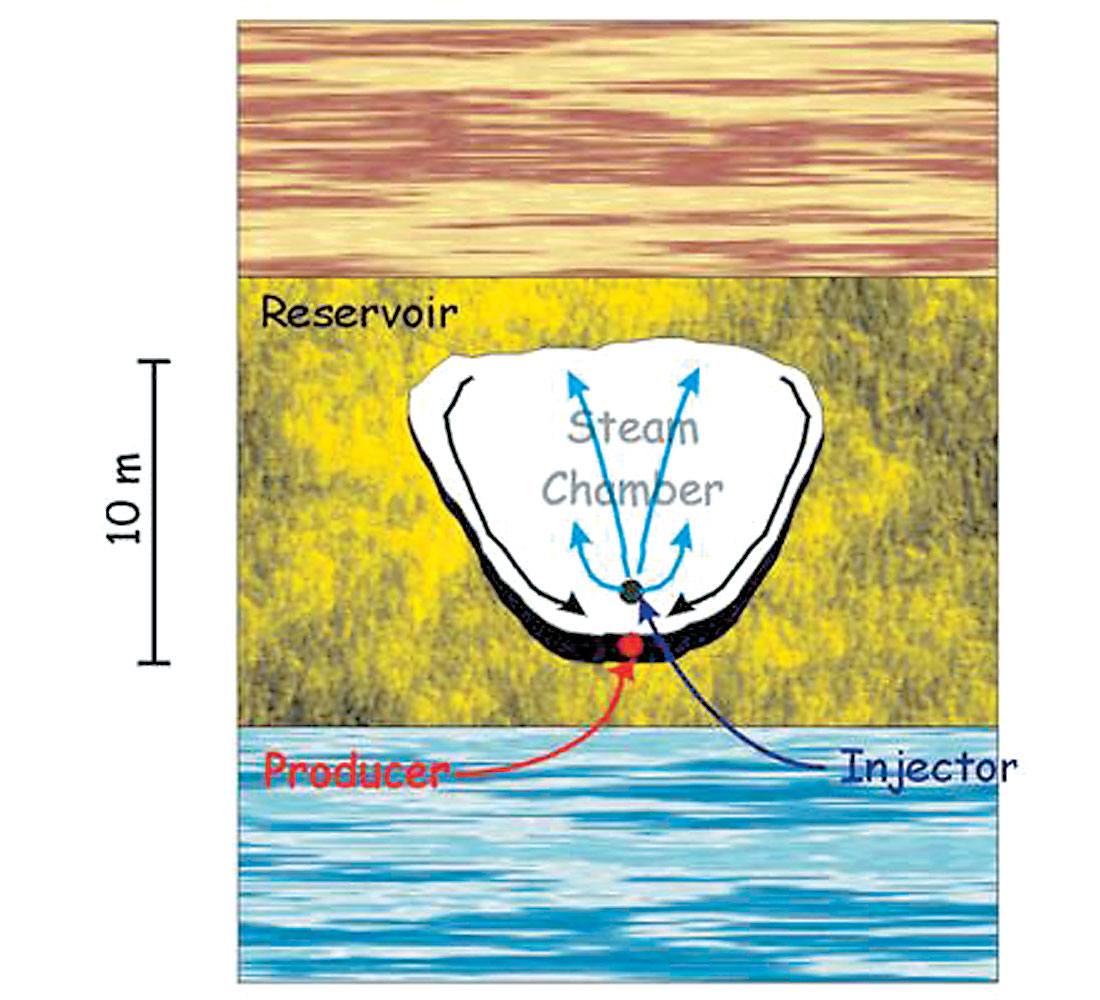
None of these methods of production is perfect. Many issues can impact the success of a project. Geologic structures within the reservoir, such as thin but impermeable shale stringers can impede and direct the flow of steam or solvents. Sanding of wellbores can block the injection or recovery. Improper wellbore completions can damage the injector or producer wellbores such that sections of the reservoir are bypassed in the production. Engineers often can only infer that there are problems on the basis of unexpected temperatures, production rates, and anomalous reservoir pressures. Even when they have found a problem they usually cannot know where along a horizontal wellbore the problem exists, and, further, it is difficult on the basis of this knowledge to know what the lateral extent of the problem might be. For these reasons, to the first order, geophysical remote sensing of the production is useful to delineate zones of bypassed reservoir. From there, geophysical methods could have added use in constraining the actual conditions within the reservoir.
These problems have motivated a number of geophysical field studies, many of which have been conducted in Canada, and a few of these are mentioned here. Pullin et al., (1987), Paulsson et al., (1994), and Schmitt (1999) describe seismic measurements in typical Athabasca reservoirs. Macrides et al (1988), Kalantzis et al (1993), Eastwood (1994), and Siewert (1994) discuss measurements in Cold Lake oil sands. Li et al. (2001) and Zhang and Schmitt (2002, 2004) describe seismic time lapse measurements at a typical Lloydminster-type reservoir while Chen et al. (2003) showed seismic responses due to cold production.
Seismic Rock Properties
The production of any reservoir requires that the in situ conditions change, and this is particularly true for heavy oil production where injection of heated fluids results in extrinsic changes in pressures and temperatures. Reservoir (i.e. pore fluid) pressures both increase and decrease during a production cycle; this can lead to the absorption or the exsolution of gas from solution. The temperature will obviously be influenced greatly by the heat input to the reservoir. Both the flow of fluids and heat can generate additional total stresses to the rock frame. The reservoir rocks may also be irreversibly changed by the variations in conditions within the reservoir. All of these will affect the elastic, and hence seismic, properties of the in situ rocks. Many of these changes have been discussed in the literature in the past but will only be summarized briefly here in the influence table of Figure 4. However, the influence of effective pressure (Pc-Pp) and saturation state on VP and VS was first examined by Domenico et al, 1977 (Figures 5-6). Timur (1977) studied the influence of temperature on rock frame velocities while heavy oil reservoir fluids and cores were initially described by Wang and Nur (1988) and for Cold Lake cores by Eastwood (1993).
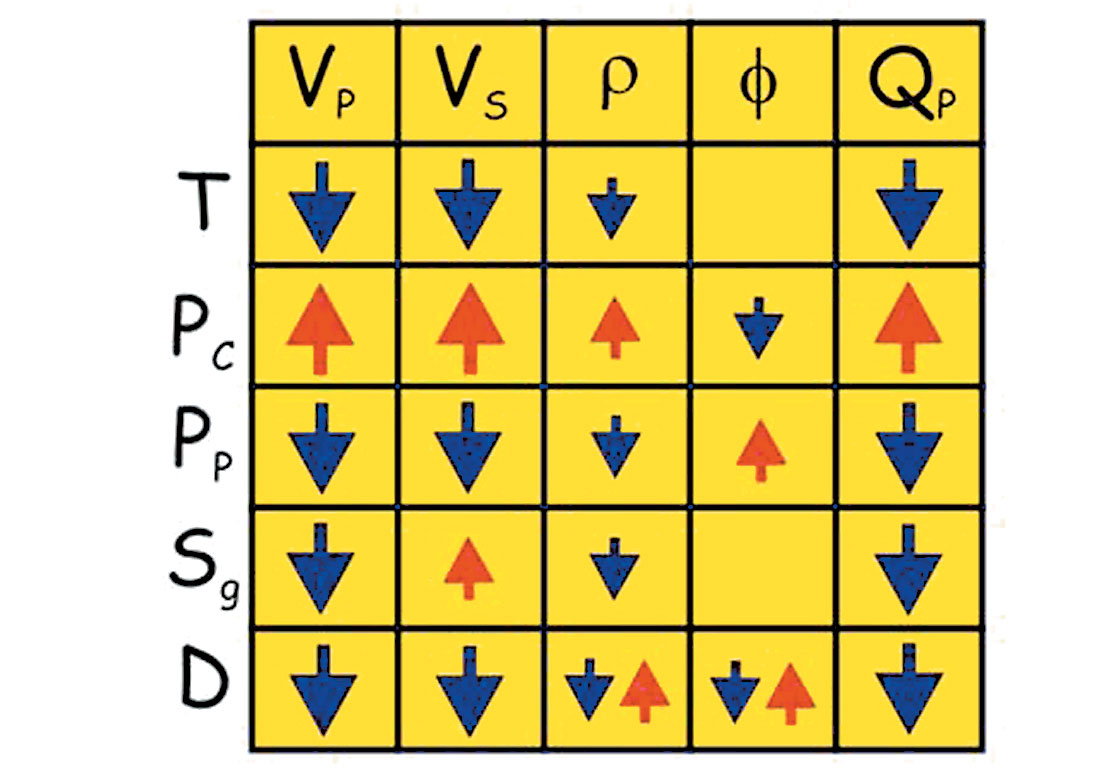
This influence table means to show that there are many factors that will influence the overall elastic properties of reservoirs in general and heavy oil reservoirs in particular. In time lapse monitoring of a reservoir, one hopes for a sufficient change in the seismic impedance rVP of the material such that the reflectivity or travel times or both are changed enough to be detected. Many reservoir scenarios are available however, and a variation in one condition can have the opposite effect with different properties. In one example, increasing pore pressure will generally result in a lower VP due to declining effective pressure, but if the pore pressure reaches the dew point then any free gas will go back into solution such that the velocity of the overall fluid-saturated rock will increase.
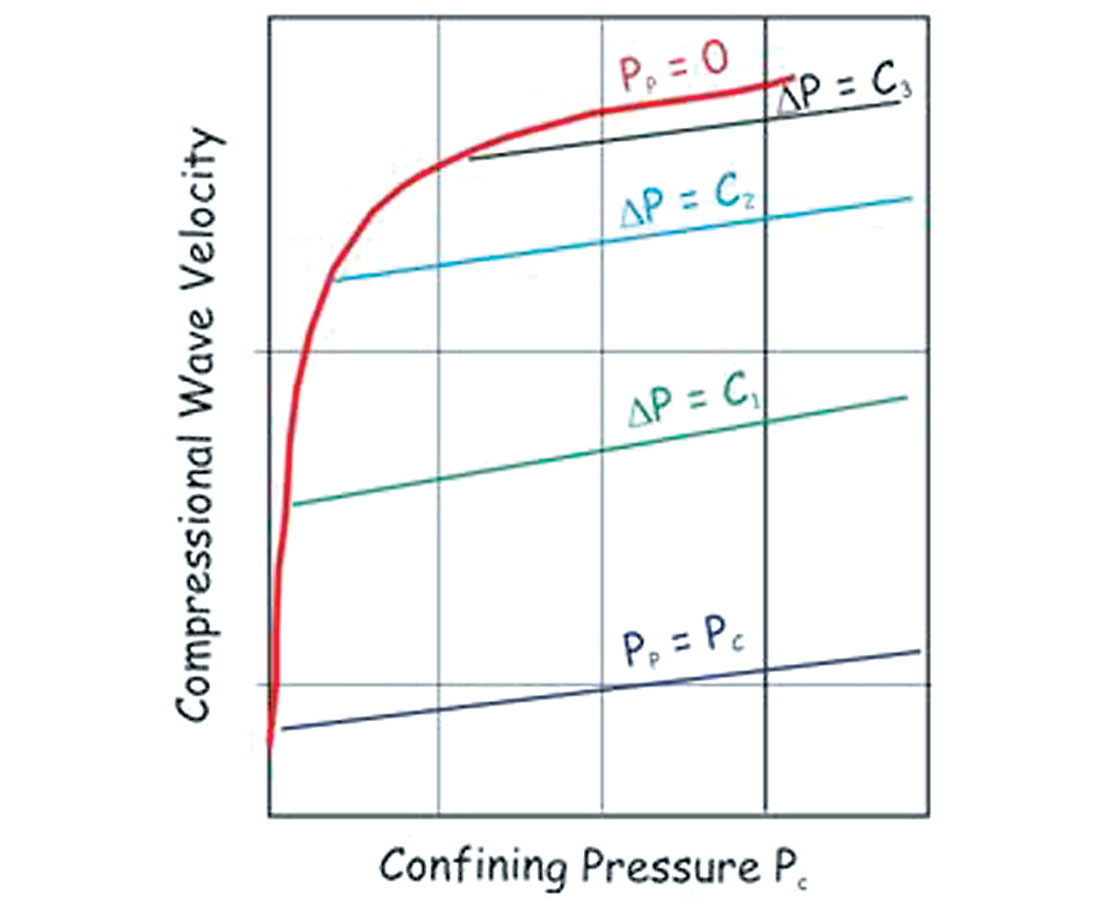
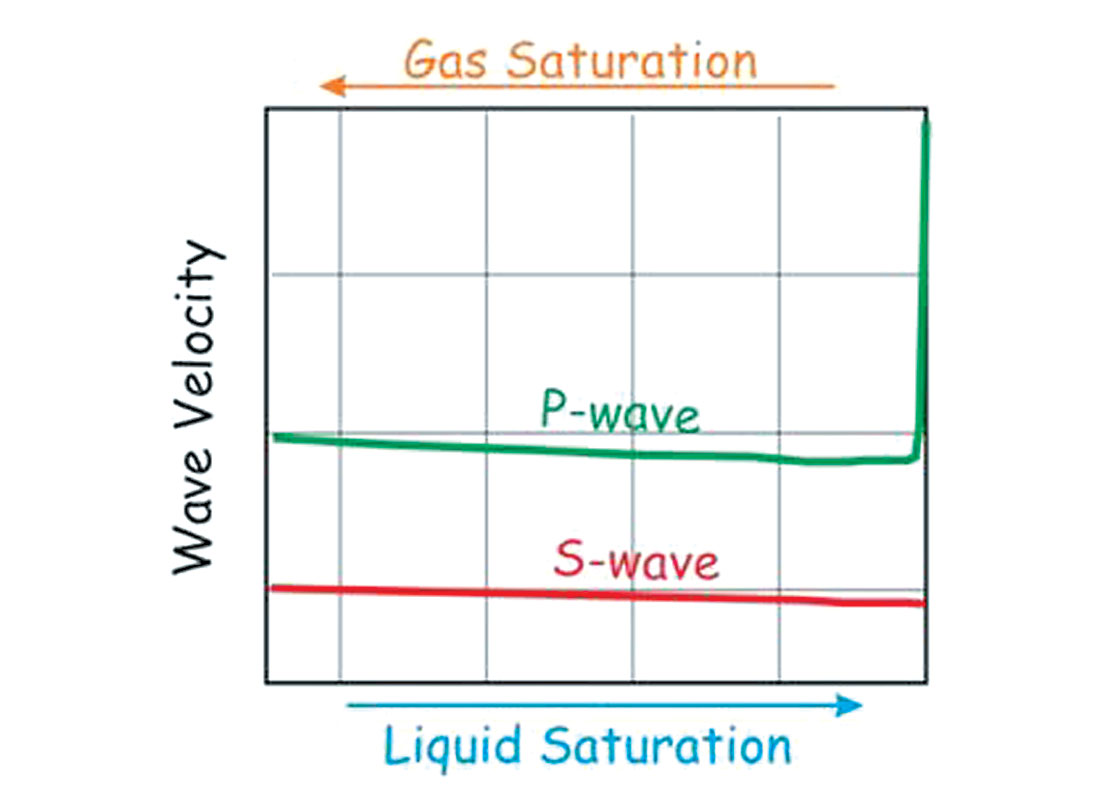
Consequently, the conditions that could lead to an observable response include but are not restricted to:
Increased pore pressure: Will lower the velocity unless free gases (e.g. methane or steam) reach the dew point and condense or are absorbed. Conversely, as pore pressure decreases the bubble point is reached such that free gas is exsolved from solution; it is likely that it is this release of free gas with declining reservoir pressure, for example, that accounts for the relatively strong seismic response in cold production.
Increased temperature: Will generally lower the velocity of both the rock frame and the pore fluids. This change can be on the order of 25% for heavy oils over temperature ranges typically encountered in reservoir stimulation.
Free gas saturation: Another point to reiterate here is that even a small amount of free gas will influence the overall compressional wave velocity substantially. However, this effect will depend critically on the overall stiffness of the rock frame. Consequently, the largest changes are expected in relatively shallow reservoirs with highly compressible unconsolidated rock. Gas-for-liquid substitution effects are likely to be more muted in deeper reservoirs which are subject to higher confining pressures tending to make the rock frame stiffer (Theune and Schmitt, 2004).
Current Research
As noted, there have already been a number of studies on the utility of time lapse measurements in relation to heavy oil production discussed in the open literature. One interesting anecdotal observation by this writer that suggests that such studies are moving to a different stage in development is that there are now many proprietary studies underway by various producers in many different geological scenarios. That the results of such surveys are not being publicly presented is possibly in part due to the recognition that such surveys are seen to have economic value.
Despite this some large tasks remain ahead of us if we are to ‘add value’ to seismic observations. One would hope to be able to tie the seismic observations to a better understanding of what the current conditions are within the reservoir in order that engineers are able to make informed decisions. This further must be done on a timely basis. A basic work flow for interpreting time lapse results would include a motion of information between reservoir simulation, the integration of such results with appropriate rock physics in order to create realistic seismic models, and finally the interpretation of the field seismic observations. Some issues that need further work and which are currently limiting progress in this area are:
- The lack of a strong understanding of the behavior of the physical properties of unconsolidated heavy oil reservoirs under changing conditions of pressure, temperature, and saturation. This will require some basic work in measuring heavy oil properties themselves, in upscaling the results of laboratory measurements to seismic scales, and in converting such information to parameters that are useful to geologists and petroleum engineers.
- How to integrate rock physics observations into reservoir simulations. One major problem here is that practical computational constraints make the cell dimensions used in reservoir simulations relatively large.
- Seismic modeling that can include the finer scales (~100 m) of typical time lapse observations over steam injection.
A brief summary of some of the work currently underway at the Universities of Alberta and Calgary includes:
- Modelling of the effect of worm holes in cold production on seismic responses. (e.g. Chen et al, 2003) and of injected steam and steam chambers (Theune and Schmitt, 2004a,b).
- Determination of the attenuation of oil sands using high resolution vertical seismic profiles (Solano and Schmitt, 2004).
- Tests of seismic repeatability in high resolution monitoring (e.g. Zhang and Schmitt, 2003).
- Understanding the effective pressure behavior of weakly consolidated sandstones as shown in Figure 7. (e.g. He and Schmitt, 2004).
- Better understanding of what actually binds the grains of sand together in ‘unconsolidated’ reservoirs. Is the assumption that such water grains are water wet universal or can the heavy oils themselves act as a cementing agent (Figure 8) (Rokosh and Schmitt, 2004).
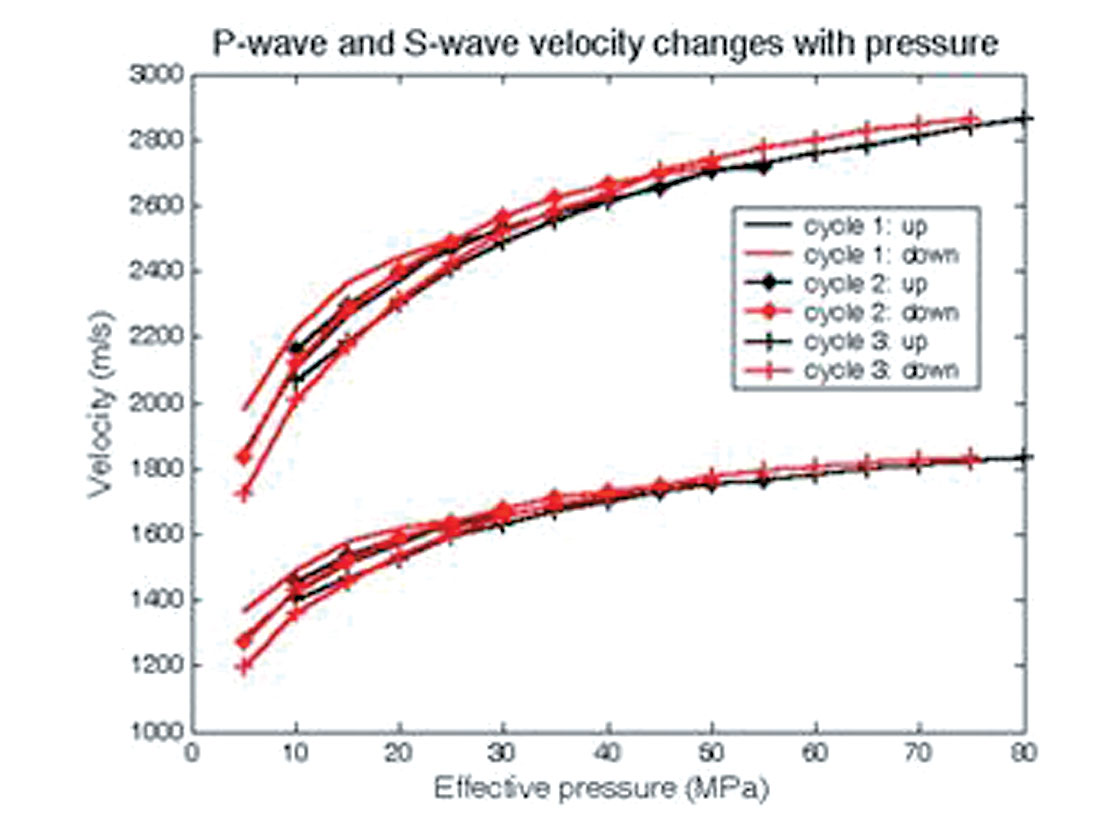
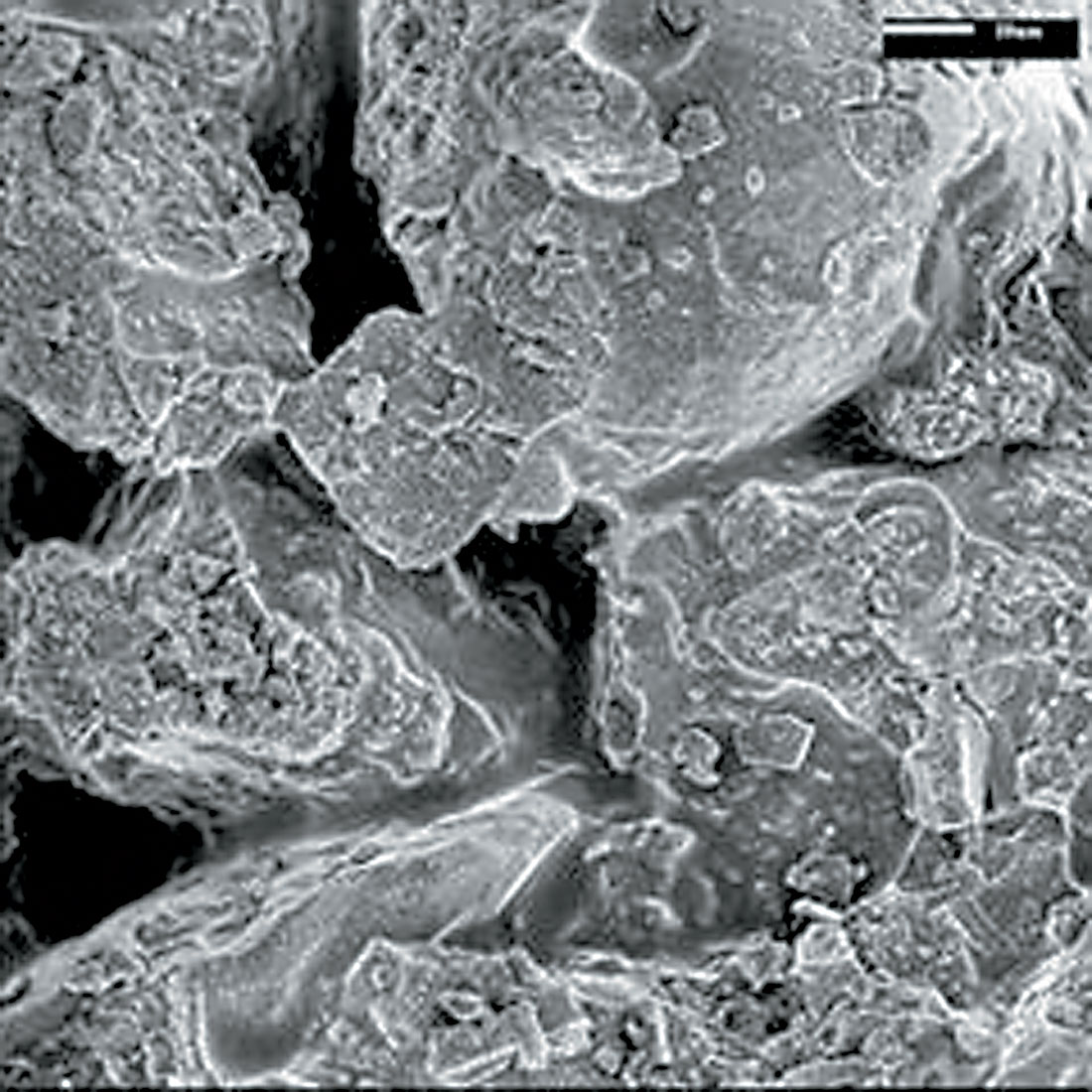
Concluding remarks
The complexity of heavy oil reservoir materials makes them interesting to study. Although there has been a good deal of initial work, there remain many challenges that remain to be tackled in understanding the behavior of reservoir materials under in situ conditions and in using such information to interpret seismic observations. Heavy oil reservoirs are also good laboratories to test concepts in time lapse monitoring. As such, it is likely that we will be seeing many new developments from this growing area of the Canadian petroleum industry in the next few years.
Join the Conversation
Interested in starting, or contributing to a conversation about an article or issue of the RECORDER? Join our CSEG LinkedIn Group.
Share This Article