Introduction
It has been recognized in recent years that ongoing methane production is occurring in deep coal beds. This observation was deduced from studies of isotopic fractionation of coal gases, changes in coal-gas chemistry and the biodegraded nalkane pattern of some coal-extract samples (Scott, 1999). Research is limited in understanding the significance of this secondary biogenic gas production. Questions are being raised as to whether the microorganisms involved in methanogenesis could be manipulated and exploited to enhance and increase the methane recovered during CBM operations. The possibility and potential of microbially enhanced CBM is being studied at the Alberta Research Council (ARC).
The ARC’s enhanced coalbed methane research program encompasses different methods for enhancing methane recovery from deep, unmineable coal beds. These include acid gas and flue gas injection as well as CO2 injection (Gunter et al., 1997). See Table 1 for publications by ARC on this topic. This last process involves injecting CO2 into deep, unmineable coal beds. When the CO2 absorbs into the coal it displaces the coalbed methane. The potential for storing CO2 in coal beds is great and represents a significant greenhouse gas abatement technology. It is well known that there are many methanogenic bacteria in nature which can convert CO2 to methane. It is, therefore, possible that coalbed-sequestered CO2 could be biologically converted to methane, thereby closing the fuel cycle in a sustainable manner.
ARC has formed a research consortium to study and develop microbial methane production from coal and sequestered CO2. The consortium members to date are: Dr. Bill Gunter, ARC (CO2 storage and enhanced CBM recovery); Dr. Karen Budwill, ARC (microbiology, methanogenesis); Dr. Marc Bustin, University of British Columbia (coalbed methane, field trials); Dr. Karlis Muehlenbachs, University of Alberta (isotopic fractionation analysis of coal bed gases); and Dr. Maurice Dusseault, University of Waterloo (engineering, injection of biosolids into deep subsurface, fracturing).
The potential for greatly increasing the methane recovered from coal alone by microbially enhanced CBM is speculated to be vast. An increase of approximately 16% of the current US coalbed methane reserves (excluding Alaska) could be generated if only one-hundredth of 1% of the coal were converted into methane by microbes (Scott, 1999).
Research in this area is, however, still in its infancy and much must be learned about the limitations to microbial growth in coal beds.
But if the process is proven to be economically feasible, microbially enhanced coalbed methane can expand the life and value of coal beds and play a role in CO2 sequestration. (See page 42 for Table 1: CO2 enhanced coalbed methane work done by the Alberta Research Council Note: papers are grouped by subject and numbered from 1 to 36 based on date of publication.)
(See Table 1: CO2 enhanced coalbed methane work done by the Alberta Research Council Note: papers are grouped by subject and numbered from 1 to 36 based on date of publication.)
Table 1: CO2 enhanced coalbed methane work done by the Alberta Research Council Note: papers are grouped by subject and numbered from 1 to 36 based on date of publication. | |
Geological Options for CO2 Storage16. Sedimentary Basins and Greenhouse Gases: A Serendipitous Association, Brian Hitchon, W.D.Gunter, Thomas Gentzis, Roger Bailey. Energy Convers. Mgmt. 40, 825-843 (1999). 15. Storage Capacity of CO2 in Geological Media in Sedimentary Basins with Application to the Alberta Basin, Stefan Bachu and W.D.Gunter. Proceedings of the 4th International Conference on GHG Control Technologies, Interlaken, Switzerland, (Editors: P.Reimer, B. Eliasson, A. Wokaun), Pergamon, 195-200 (1999). 12. Large CO2 Sinks: Their Role in the Mitigation of Greenhouse Gases from an International, National (Canadian) and Provincial (Alberta) Perspective, W.D.Gunter, S.Wong, D.B.Cheel, G.Sjostrom. Applied Energy 61, 209-227 (1998). 5. Carbon Dioxide Disposal. S.Bachu, W.D.Gunter and E.H.Perkins, Chapter 3 in Aquifer Disposal of Carbon Dioxide: Hydrodynamic and Mineral Trapping - Proof of Concept, Editor: B.Hitchon, Geoscience Publishing Ltd., Sherwood Park, Alberta, p.11-22 (1996). Enhanced Oil Recovery13. Injection of CO2 for Enhanced Energy Recovery Coalbed Methane versus Oil Recovery, Sam Wong:, Chris Foy, Bill Gunter and Tom Jack. Proceedings of the 4th International Conference on GHG Control Technologies, Interlaken, Switzerland, (Editors: P.Reimer, B. Eliasson, A. Wokaun), Pergamon, 189-194 (1999). Enhanced Coalbed Methane Recovery31. Biogenic Methane Production from Coal with Implication for Carbon Dioxide Sequestration, Karen Budwill, Andrew Beaton, Marc Bustin, Karlis Muhlenbachs and W.D. Gunter, Proceedings of the 6th International Conference on GHG Control Technologies, Kyoto, Japan (in press) 28. Testing for CO2 Sequestration and Enhanced Methane Production from Coal, M. J. Mavor, W..D.Gunter, J.R. Robinson, D. H-S. Law and John Gale, SPE paper 75680, Presented at the SPE Gas Technology Symposium, May 30-April 2, Calgary, 14 p (2002) 26. CO2-Enhanced Coalbed Methane Recovery Demonstration Pilot- A Case for Australia, S. Wong, K. MacLeod, M. Wold, W.D. Gunter, M.J. Mavor and J. Gale, Proceedings of the 2001 International CBM Symposium, Tuscaloosa, USA, May 14 to 18, 75-86 (2001). 25. Site Ranking for CO2-Enhanced Coalbed Methane Demonstration Pilots, S. Wong, W.D. Gunter, and J. Gale, Proceedings of the 5th International Conference on GHG Control Technologies, Cairns, Australia, (Editors: D. Williams, B. Durie, P. McMullan, C. Paulson, Andrea Smith) CSIRO Publishing, 531-536 (2001). 23. Economics of Flue Gas Injection and CO2 Sequestration in Coalbed Methane Reservoirs, S. Wong, W.D. Gunter, D. H.-S. Law and M.J. Mavor, Proceedings of the 5th International Conference on GHG Control Technologies, Cairns, Australia, (Editors: D. Williams, B. Durie, P. McMullan, C. Paulson, Andrea Smith) CSIRO Publishing, 543-548 (2001). 22. Enhanced Recovery of Coalbed Methane with Carbon Dioxide Sequestration- Selection of Possible Demonstration Sites, International Energy Agency Greenhouse Gas R&D Programme Report Number PH3/34, 178p. (2000). 19. Economics of CO2 Sequestration in Coalbed Methane Reservoirs, S. Wong, W.D. Gunter, M.J. Mavor. Proceedings of SPE/CERI Gas Technology Symposium 2000, SPE 59785, April 3-5, Calgary, Alberta, 631-638 (2000). |
18. Updating Canadian Activities in Coalbed Methane, Ken Sinclair, W.D. Gunter, S. Wong. Proceedings of the Coalbed and Coal Mine Methane Conference in Denver, March 27-28, 9 pages (2000). 11. Deep Coalbed Methane in Alberta, Canada: A Fuel Resource with the Potential of Zero Greenhouse Gas Emissions, W.D.Gunter, T.Gentzis, B.A.Rottenfusser and R.J.H. Richardson, Energy Convers. Mgmt. 38 Suppl., S217-S222 (1997). Industrial Analogues for Geological Storage: Acid Gas Reinjection35. Acid Gas Re-injection: An Innovative CO2 Storage Opportunity, Stefan Bachu and W.D. Gunter, in Geological Storage of Carbon Dioxide for Emissions Reduction, Geol.Soc. of London Spec. Pub. (in press) 32. Economics of Acid Gas Reinjection: An Innovative CO2 Storage Opportunity, Sam Wong, David Keith, Edward Wichert, Bill Gunter and Tom McCann, Proceedings of the 6th International Conference on GHG Control Technologies, Kyoto, Japan (in press) Capture of CO230. Surface Facilities Computer Model: An Evaluation Tool for Enhanced Coalbed Methane, Doug Macdonald, Sam Wong, W.D. Gunter, Rick Nelson and Bill Reynen, Proceedings of the 6th International Conference on GHG Control Technologies, Kyoto, Japan (in press) 20. Recovery of CO2 from Flue Gas, CO2 Sequestration, and Methane Production from Coalbed Methane Reservoirs, J. Ivory, W.D. Gunter, D. Law, S. Wong and X. Feng, Proceedings of the International Symposium on Ecomaterials, Ottawa, Canada (Editor: H. Mostaghaci), Canadian Institute of Mining, Metallurgy and Petroleum, Montreal, 487-501 (2000). 17. Geological Storage for CO2: Options for Alberta, S. Wong, W.D. Gunter and S. Bachu. Presented at Combustion Canada 99, Calgary, May 26- 28, 12 pages (1999). Enhanced Coalbed Methane Recovery: Numerical Model Comparison34. Comparison of Numerical Simulators for Greenhouse Gas Storage in Coalbeds, PartII: Flue Gas Injection, D,H,-S. Law, L.H.G. van der Meer and W.D. Gunter, Proceedings of the 6th International Conference on GHG Control Technologies, Kyoto, Japan (in press) 29. Numerical Simulator Comparison Study for Enhanced Coalbed Methane Recovery Processes: Part I Pure CO2 Injection. D. H-S. Law, W.D. Gunter and L.G.H. van der Meer, SPE 75669, Presented at the SPE Gas Technology Symposium, May 30-April 2, Calgary, 12p (2002) 27. Comparison of Numerical Simulators for Greenhouse Gas Sequestration in Coal Beds Part I: Pure Carbon Dioxide Injection, D.H.-S. Law, L.G.H. van der Meer, and W.D. Gunter, Proceedings of 1st National Conference on Carbon Sequestration, Washington, DC., May 14-17, 15 p. (2001) 24. Modelling of Carbon Dioxide Sequestration in Coal Beds: A Numerical Challenge, D.H.-S. Law, L.G.H. van der Meer, M.J. Mavor and W.D. Gunter, Proceedings of the 5th International Conference on GHG Control Technologies, Cairns, Australia, (Editors: D. Williams, B. Durie, P. McMullan, C. Paulson, Andrea Smith) CSIRO Publishing, 537-542 (2001). Contact: William D. Gunter, Ph.D., Carbon and Energy Management, Alberta Research Council, 250 Karl Clark Road, Edmonton, Alberta, Canada T6N 1E4. Tel: (780) 450-5467; Fax: 450-5083; Email: gunter@arc.ab.ca, Website: www.arc.ab.ca; -April, 2003- |
Methanogenesis
Methane can be produced by either thermogenic or abiogenic formation occurring in subsurface carbon deposits or by biological or biogenic formation occurring usually at or near the earth’s surface (Tyler, 1991). Biogenic methane is the result of complex biochemical reactions by groups of bacteria during the decomposition of organic matter in anoxic environments. Three functionally different trophic groups of bacteria are required for methanogenesis (Figure 1). These include 1) hydrolytic fermentative bacteria, 2) syntrophic acetogenic bacteria, and 3) methanogenic bacteria (Boone, 1991). Fermentative bacteria initially hydrolyze complex organic compounds to acetate, longer chained fatty acids, CO2, H2, ammonia (NH3) and hydrogen sulphur (HS-). The activities of syntrophic acetogenic bacteria are crucial to complete methanogenic fermentation. Low molecular weight fatty acids such as propionate and butyrate are common products of fermentation bacteria. These acids, however, cannot be catabolized by methanogens. The syntrophic acetogenic bacteria oxidize these acids to acetate (and CO2 in the case of propionate) producing H2 or formate as the reduced product. The principle substrates, therefore, used by methanogenic bacteria to promote their growth include hydrogen (H2), CO2, acetate and formate. Methanogens are a group of strict anaerobic archaebacteria requiring reducing environments (redox levels Eh < -200 mV) for growth and produce methane as the final product. The most common and almost universal mode of methane production is via the H2-mediated reduction of CO2 (carbonate reduction pathway, Figure 1), although the major source of methane in most environments active in organic decomposition, such as anaerobic digesters and freshwater sediments, is acetate (fermentation pathway, Figure 1) (Jones, 1991).
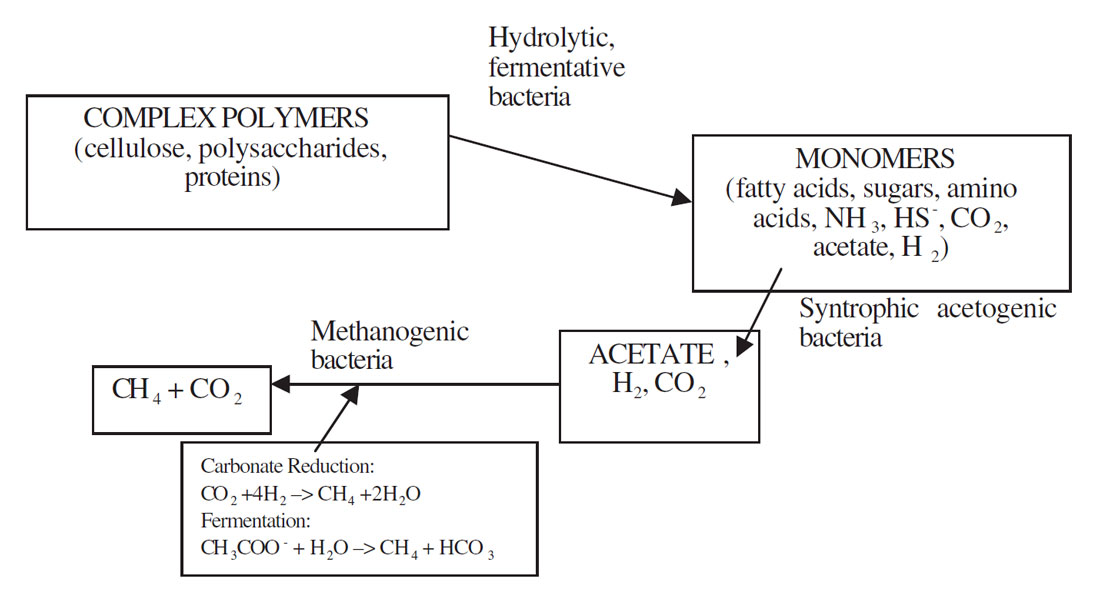
Environmental factors such as pH, salinity, available inorganic and organic nutrients, and toxic substances can all affect methanogenesis (Boone, 1991). Low pH conditions and accumulated acids are thought to be more inhibitory to methanogens then to fermentative bacteria. The production of fermentation acids causes a drop in the pH and subsequently inhibits the ability of methanogens to remove these acids. The optimal temperature for most mesophilic methanogens is 35°C. With a decrease in temperature there is a decrease in the metabolic rate. It had been found that low salinities in the range of 0 to 0.2 M NaCl have minimal effects on the growth rates of adapted methanogenic consortia but higher salinities are inhibitory.
Environmental conditions also exert their effect on the consortia of degradative bacteria of which methanogens are a part. In environments with slow rates of organic matter turnover, it is generally the hydrolysis of large polymers which limits microbial activity in anoxic environments and low-molecular-weight intermediates do not accumulate appreciably (Boone, 1991). Almost all organic molecules are biodegradable under anoxic conditions, although resistance to degradation varies. Recalcitrance is enhanced by polymerization and by the presence of substituents such as alkyl branches, halogens, or nitro- and sulfo-groups.
Coal Biodegradation and Methanogenesis
Coals are composed of an interconnected network of hydroaromatic and cycloalkanes, with chemical linkages such as ethers and C-C bonds that are not easily hydrolyzed by anaerobic bacteria (Deobald, 1991). The conversion of coal to a lower molecular weight product has been demonstrated using mostly aerobic microorganisms (Catcheside and Ralph, 1999). However, investigations on similar compounds to coal or individual compounds that can make up coal suggest there is potential for depolymerization of coal by anaerobic bacteria (Deobald, 1991).
Studies on the biological processing of coal (Catcheside and Ralph, 1999) showed there are two possible mechanisms of biosolubilization of coal. In the first mechanism, microorganisms growing on rich media generate alkaline metabolic products that lead to the ionization of acidic groups in low-rank coal. This causes coal humates to become water-soluble. Investigations by Shumkov et al. (1999) showed how the addition of enclosing alkaline rocks might affect the efficiency of biogas production from coals. The enclosing rocks (consisting of clay minerals, carbonate, feldspar and quartz with between 17-26% organic matter) may have served as additional sources of essential micro-and macro-elements for indigenous microorganisms. The pH of the medium or surrounding area could also have changed due to the presence of alkaline rocks. The addition of the rocks raised the pH of the incubation medium causing the transfer of cations from the medium to coal surface to increase leading to an increase in methane production. It was found that the addition of coals to water solutions caused an ion exchange between the water phase and the coal. Alkaline rocks, alkalineearth metals and H+ ions participated in this exchange. The degree of abiotic transformation of coal (by alkaline solubilization) was found to correlate with the initial pH of the incubation medium with maximal solubilization occurring at high pH values of 8-10 (Shumkov et al., 1999).
The other mechanism of coal biosolubization involves the production of oxalate by microorganisms (Catcheside and Ralph, 1999). Oxalate acts as a chelater to sequester polyvalent metal ions, such as Fe3+, Mg2+ and Ca2+, present in coal and involved in ionic linkages in coal humates. The removal of these metal ions by sequestration increases the solubility of the coal. This results in the removal of ionic links between structural elements and an increase in the numbers of free acidic groups.
High-rank coals were shown to support methanogenic growth by Johnson et al. (1994) and Volkwein et al. (1994). Cultures consisting of different anaerobic consortia believed to have significant potential for degrading coal were tested for methane production on three different coal types. At least three consortia (anaerobic sewage sludge, mine treatment sludge, and oil/chemical waste) were isolated from natural sources capable of anaerobically converting at least portions of higher-rank coals to methane. The methane yields were relatively low, between 2-5% methane, but greater than control cultures containing no coal.
Panow et al. (1997) also investigated the microbial conversion of coal to methane using different anaerobic environments as sources for methanogenic consortia. Loadings of coal refuse fines greater than 8% (w/v) were found to be inhibitory to gas production. It is possible that the trophic levels of the methanogenic consortia became unbalanced, and that some bacteria were inhibited while others were not (Panow et al., 1997). This observation supports previous claims that coal is a better inhibitor of microbial growth than it is a substrate. While more methane was produced in coal cultures than from coal-free controls, there was a negligible reduction in the mass of the coal in the cultures and a significant discrepancy between observed and predicted methane and CO2 production based on stoichiometric equations. It was suggested that perhaps a non-microbial source of methane may be masking the actual methane that originated from the bacterial conversion of coal. This would indicate that the contribution to the amount of observed methane made by the microbial conversion of black coal is minor.
Results, however, from laboratory microcosms done by our group at ARC concur with the results observed by Johnson et al. (1994) and Volkwein et al. (1994). We also observed a slight increase in methane yields (approximately 2%) over non-coal control cultures when sewage sludge was the source of methanogenic bacteria. Coal rank, and coal chemistry in particular, affected biogenic methane production as inhibitory effects were observed in cultures given coal with acidic properties.
Deep Subsurface Microbial Communities
Deep subsurface communities have only recently been identified by using sophisticated recovery/sampling and tracer methods. These communities are believed to be supported by organic matter that was either deposited with the formation sediments or which migrated from the surface along groundwater flow paths (Krumholz et al., 1997).
One of the most all-encompassing attempts to isolate microorganisms from coal was the work done by Polman et al. (1993) on Argonne Premium coal samples. The researchers looked at the microbial content of different lots of three Argonne Premium coals (lignite, sub-bituminous, and bituminous coals) using 28 kinds of growth medium incubated under aerobic, microaerobic, and anaerobic conditions. The microorganisms targeted were aerobes, microaerophiles, facultative and strict anaerobes, fungi, sulfate-reducing bacteria, methanogens and chemoheterotrophs. Their results from growth studies looking at changes in culture turbidity and production of methane showed no activity in any of the cultures. Since no viable microorganisms were derived from the coal samples, the coals were deemed pristine. The authors hypothesized that perhaps there was antimicrobial activity associated with the coal. They also conceded that since they started with a small amount of coal in their cultures (5% w/v), perhaps low initial numbers and frequency of microorganisms in the Argonne coals became diluted and thus difficult to detect and culture.
Techniques for sampling and isolating microorganisms from environmental samples, in particular those from the deep subsurface, have improved since the work by Polman et al. (1993) (Griffen et al., 1997). A larger sample size and longer incubation periods would have perhaps produced viable and detectable cultures in their study. It is extremely difficult to isolate and grow environmental cultures due to difficulties in mimicking environmental growth requirements and conditions in the laboratory. Modern molecular biology techniques eliminate the need to actually grow the environmental sample in prepared culture media, and are used to demonstrate the presence of microorganisms in the deep subsurface (Sorensen et al., 2002). Molecular biology techniques can also be used to genetically modify the isolated organism in order to improve certain traits of that organism, such as increased or enhanced enzymatic activity.
Microbially Enhanced Coalbed Methane: Proposed Technology
Microbially enhanced coalbed methane involves the introduction of microorganisms and/or nutrients and/or trace elements into the coal bed in order to stimulate and enhance CBM production. The injection of just nutrients and/or trace elements to enhance indigenous coal bed methanogenic consortia can also be performed. Figure 2 is a schematic drawing of the possible reactions and pathways that can occur in a CBM recovery operation when microorganisms are introduced or indigenous microorganisms stimulated. The different trophic levels of microorganisms produce from coals the substrates required by methanogens to make methane.
The delta 13C of methane in the deep subsurface is commonly measured to determine whether the gas is biogenic or thermocatalytic in origin. Thermogenic methane is generally, but not exclusively, enriched in 13C compared with bacterial methane (Whiticar, 1999). Indeed, microbial methane represents the most 13C-depleted substance on Earth (Grossman, 1997). These isotopic ratios vary because kinetic processes such as bacterial reactions preferentially use the lighter isotope of an element due to a lower activation energy for bond breaking and because isotopic exchange occurs between different chemical substances, different phases, or individual molecules as chemical processes move toward isotopic equilibrium. It is also possible to distinguish between acetate fermentation and bacterial carbonate reduction. In bacterial carbonate reduction the methane is generally more depleted in 13C and enriched in D (Whiticar, 1999). There are several lines of evidence suggesting that carbonate reduction is probably the preferred metabolic pathway in coal beds (Scott, 1999). Bacteria derive nearly all of the hydrogen from formation water to produce methane. Acetate fermentation may also occur in some coal beds, but may not be the dominant metabolic process in the subsurface. Three hydrogen atoms in methane come from organic matter (the methyl group) and only one hydrogen atom comes from formation water.
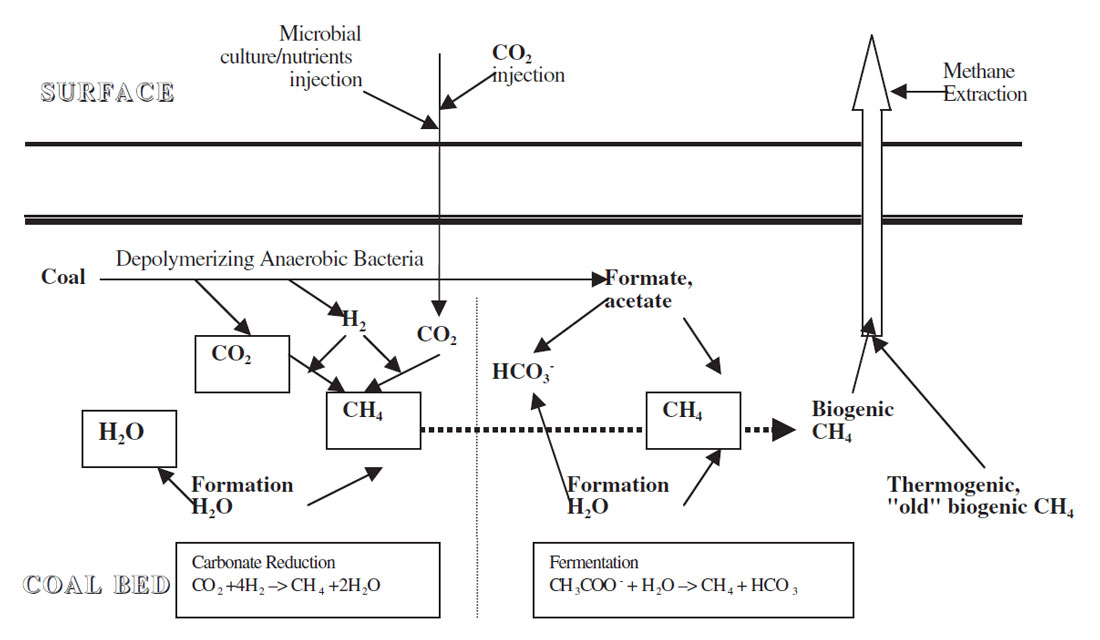
The concept of the microbial conversion of sequestered CO2 to methane in coalbeds is new and there has been, as of yet, very little reported on this in the literature. With the addition of CO2 to the coal beds, it is assumed that the indigenous methanogenic consortium or injected consortium would be able to convert the CO2 to methane as indicated in Figure 2. Access of the microorganisms to the sequestered CO2 as well as stoichiometric amounts of the reducing equivalent, hydrogen would be necessary. Hydrogen could come from the formation water (Scott, 1999) or the coal.
Benefits and Limitations to Microbially Enhanced Coalbed Methane
The most obvious benefit of microbially enhanced CBM is an increase in methane production. Methane generation rates determined from laboratory cultures can be extrapolated to reflect in situ rates. The amount of methane produced by the methanogenic consortium depends on access to the coal and the surface area exposed on the coal. Since laboratory methanogenesis experiments are conducted with crushed coal having a large net surface area, one would expect higher reaction rates than if solid coal was used. Therefore, in order to extrapolate lab results to in situ coal beds, several assumptions must be made. The main ones are that 1) the reaction rates are controlled primarily by total surface area (the amount of gas generated is proportional to the surface area), and that 2) the microbial conversion of coal generates only methane and no other gases such as CO2. Table 2 summarizes laboratory methanogenic results and extrapolated methane generation rates when a 10 m coal bed covering 10 acres with a total coal tonnage of 544,226 tons is considered (Scott, 1999).
Coal sample/microbial source | Culture conditions | Lab. Methane generation rates | Extrapolated Rates (10 m coal, 10 acres, 544,226 tons) |
---|---|---|---|
Table 2. Rates of biogenic methane production from coal | |||
Scott, 1999 | Unknown | 0.98 scf/ton coal/day | 533 Mcf/day |
ARC, Bituminous coal Thermophilic methanogenic coal culture | 60°C, 80% H2, 20% CO2 atmosphere; carbonate reduction pathway utilized | 1,052 scf/ton coal/day | 572 MMcf/day |
ARC, Bituminous coal Thermophilic methanogenic coal culture | 50°C, 80% N2, 20% H2 atmosphere; carbonate reduction pathway | 41 scf/ton coal/day | 22 MMcf/day |
ARC, Bituminous coal Thermophilic methanogenic coal culture | 50°C, 100% N2 atmosphere; fermentation pathway | 28 scf/ton coal/day | 15 MMcf/day |
It is known that chemically wet gases, condensate and waxes are generated from hydrogen-rich coals during coalification and are produced from coalbed methane wells (Scott, 1999). Bacteria can use these compounds, in particular the waxes, as food sources and this may play an important factor in the survival of coal anaerobic consortia. When these compounds are removed from the coal surface, methane sorption sites are generated for methane produced by coal bed methanogens.
Coal permeability varies greatly and affects bacterial access to the coal. The estimated cleat-aperture range of coals is between seven and 30 microns (Scott, 1999). Most microorganisms range from one to three microns in diameter with ultramicrobacteria being less than 0.3 microns in diameter. Thus, microorganisms would generally be able to fit into the cleat spaces of coals. This indicates that bacteria can be injected into coal reservoirs with small cleat aperture size and be able to access the coal. Permeability can also increase as the coal is metabolized by the hydrolytic bacteria.
There are still many uncertainties and unknowns to the process that can affect the amount of methane generated. Many factors can affect microbial viability and activity in the coal bed, including surface area available for biodegradation of coal into methane as was discussed in the previous section. Another limiting factor is the build up of waste products generated by bacteria during coal biodegradation. The generation of acid by-products, for example, could lower the pH of the surrounding environment unless there is sufficient buffering capacity in the coal bed. Bacteria have often defined pH ranges for optimal growth, and if the pH falls out of that range, bacterial activity could be reduced or inhibited.
In order to consistently maintain high methane generation rates over long periods of time, methods to remove or to counteract waste products need to be developed. Re-inoculation of the bacterial consortium and/or re-injection of nutrients and trace elements will also be required in order to prevent a drop off in biogenic methane production. Table 3 summarizes the benefits and limitations to microbially enhanced coalbed methane.
Benefits | Limitations |
---|---|
Table 3: Benefits and Limitations to Microbially enhanced coalbed methane | |
Well remediation (removal of pore-plugging coal bed waxes and paraffins). | Total cleat surface area accessible to bacteria in the subsurface. |
Increase in permeability (coal removed from cleat surfaces). | Inconsistent methane production rates. |
Generation of additional methane from coal and from CO2. | Build up of microbial waste products detrimental to microbial growth and activity |
Conclusion
There is still a need to gather more data about methane generation rates from coal bed cultures; the information so far is limited to a small sample of laboratory cultures. This data would benefit the determination of whether microbially enhanced CBM would be economically viable. Preliminary results are encouraging, however. ARC is actively pursuing this area of research and several major research gaps are identified before field testing can commence:
- Characterization of indigenous microorganisms from deep coal beds. This would involve sampling and enriching for anaerobic bacteria from coal beds. The enriched cultures would then be tested for methane generation rates in the laboratory. Growth requirements and limitations to growth and activity of culture need to be determined. The end result would be information on whether the culture or single microorganism can improve CBM production and recovery.
- Detailed understanding of hydrogeologic factors affecting CBM production. There is a need for understanding coal rank, permeability, and ground water flow, among other things, and how it affects microbial growth.
- Determining whether injected CO2 could be microbially transformed into methane at economic rates.
With a multidisciplinary research team at ARC, consisting of a microbiologist, geologists, and engineers, we plan to pursue and resolve these research gaps and develop a viable process for microbially enhanced CBM recovery.
Join the Conversation
Interested in starting, or contributing to a conversation about an article or issue of the RECORDER? Join our CSEG LinkedIn Group.
Share This Article