Abstract
The increasing demand to develop renewable energy resources has driven a surge in interest in geothermal sources, both for power generation and for direct use of heat, or for a combination of both. The most economically attractive geothermal projects are those that exploit a naturally occurring, high-temperature hydrothermal reservoir. Finding such reservoirs requires a dedicated exploration effort that spans over a number of disciplines, including geology, geochemistry, and geophysics.
The geologic conditions that accommodate geothermal reservoirs also produce deposits of certain mineral components with distinct electrical conductivity properties, and thus can be detected with geophysical electrical resistivity methods. The depths of high-temperature geothermal reservoirs (a few kilometers) are generally greater than the maximum depth of investigation achievable with conventional resistivity methods. Consequently, the magnetotelluric (MT) method has become the preferred method for exploration of deep geothermal resources.
The MT method is sensitive to the electrical resistivity signatures of the main components of the geothermal system (heat source, cap rock layer, and structures that conduct fluid flow). The MT model, integrated with surface geology and geochemistry, is used to construct a conceptual model of the geothermal system. This conceptual model is subsequently used to target deep drilling and numerical resource estimation as well as selection of the appropriate energy conversion technology and projection of project economics.
Introduction
A geothermal resource is generally characterized as a reservoir constituted of a heat source and fluid phase, geologically situated in altered or structurally deformed host rocks. All these specifications make any geophysical resistivity method a primary tool for geothermal exploration. Among the resistivity methods, the magnetotelluric (MT) technique provides the unique ability to cover a large area encompassing the geothermal system, a superior depth of investigation (i.e., several thousands of meters as opposed to other resistivity methods which are generally limited to a DOI of a few hundreds of meters), and the resolution that is required to explain often very complex geological settings.
In this article MT acquisition and processing are not discussed, rather the focus is on how geothermal resources generally appear in MT models as an end product of an MT survey (i.e., we discuss how magnetotelluric methods fit into the exploration process and plan). Among the geothermal systems, high-temperature geothermal resources are used most commonly for industrial power generation or combined heat and power and represent a challenging program and large enough capital investment to justify the cost of an MT survey effort, as will be discussed in more detail in the following sections.
There are different types of geothermal resources that produce different geophysical signatures. High-temperature resources (T>~215°C) produce certain geologic sequences that are clearly identifiable by their resistivity patterns. Because high-enthalpy geothermal reservoirs are typically found at depths greater than 1 km, the magnetotelluric method is effective in identifying the different components of the geothermal system and has become a widely used geophysical tool for exploration.
The MT data are used together with other observations to build a conceptual model for the geothermal resource, which is then used for drill targeting and economic evaluation of the geothermal project.
Types of Geothermal Systems
IGA (2014) divides geothermal play types into three general categories, depending on geologic setting and heat transport mechanism. The categories are: (i) magmatic plays, where heat is transported by the convection of hydrothermal fluids heated by active or extinct vulcanism; (ii) extensional domain plays, in which there is also convection of hot fluids but the heat source is an elevated mantle caused by crustal extension and thinning rather than volcanic activity; (iii) conduction-dominated plays, where heat is stored in a static non-convecting aquifer, and the geothermal gradient is higher than the global average. The economics of geothermal development projects will depend largely on the play type, and specifically, the temperature and depth of the geothermal resource. Electric power (or combined heat and power) projects that exploit high-temperature (>215°C) reservoirs at depths between 1 and 2 km are economically competitive with renewable energy alternatives and even with conventional thermal power sources in many locations. In contrast, deeper reservoirs at lower temperatures (<150°C) require more wells to develop, and a more complex energy conversion mechanism to generate electricity, so the levelized cost of electricity from these resources is typically higher.
Magmatic Systems
Magmatic plays are the most commonly developed geothermal resource because of economic considerations. Meteoric water permeates through a volcanic edifice and is heated by a relatively shallow magmatic intrusion and ascending hot volcanic gases. The hot water becomes buoyant and mineral-rich (typically with silica, chlorides, and other minerals dissolved from the volcanic rocks), and flows upward and outward from the volcano (Figure 1). Depth to the top of the reservoir varies typically between 1 and 3 km for this type of resource, and temperatures commonly range from 215 to 310°C. Such plays are usually identified in regions with active basaltic volcanism at divergent plate margins (e.g., Iceland), basaltic to andesitic volcanism along island arcs (e.g., Indonesia), or recent andesitic to dacitic volcanism (e.g., Central America or Japan).
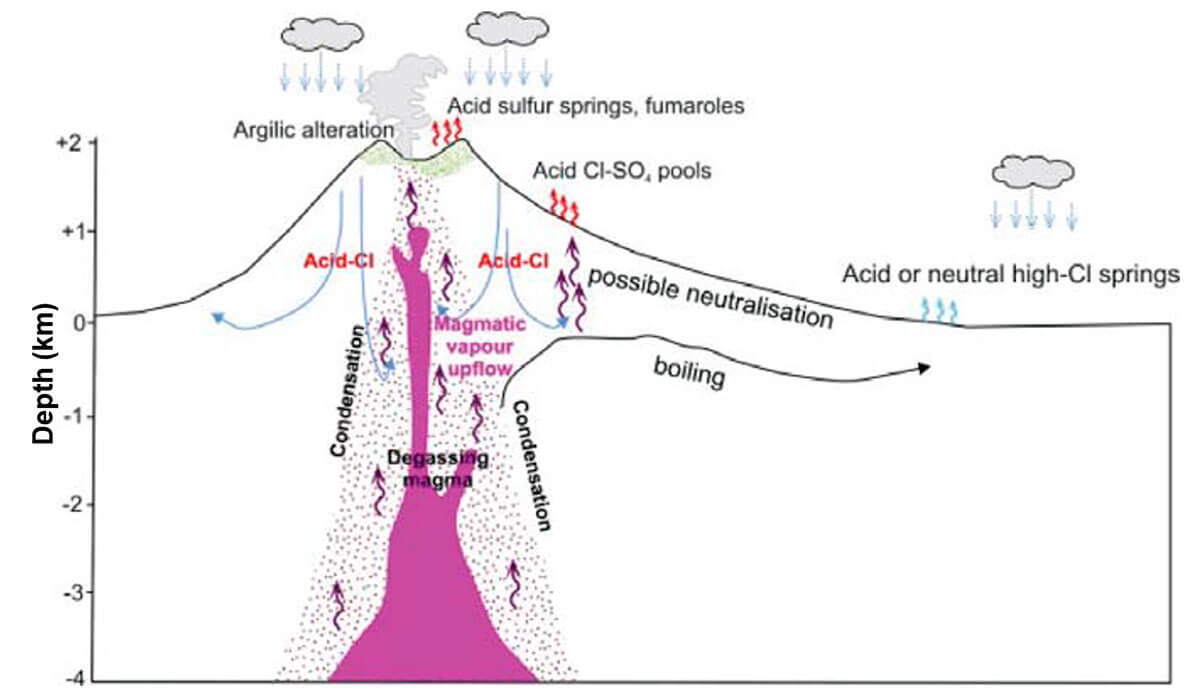
There are also magmatic plays where the volcanic intrusion does not break to the surface (Figure 2). In such plays, there may be no volcano visible nearby, as the magma chamber lies beneath flat terrain. A well-known example of this type of play is the Cerro Prieto geothermal field in Mexico.
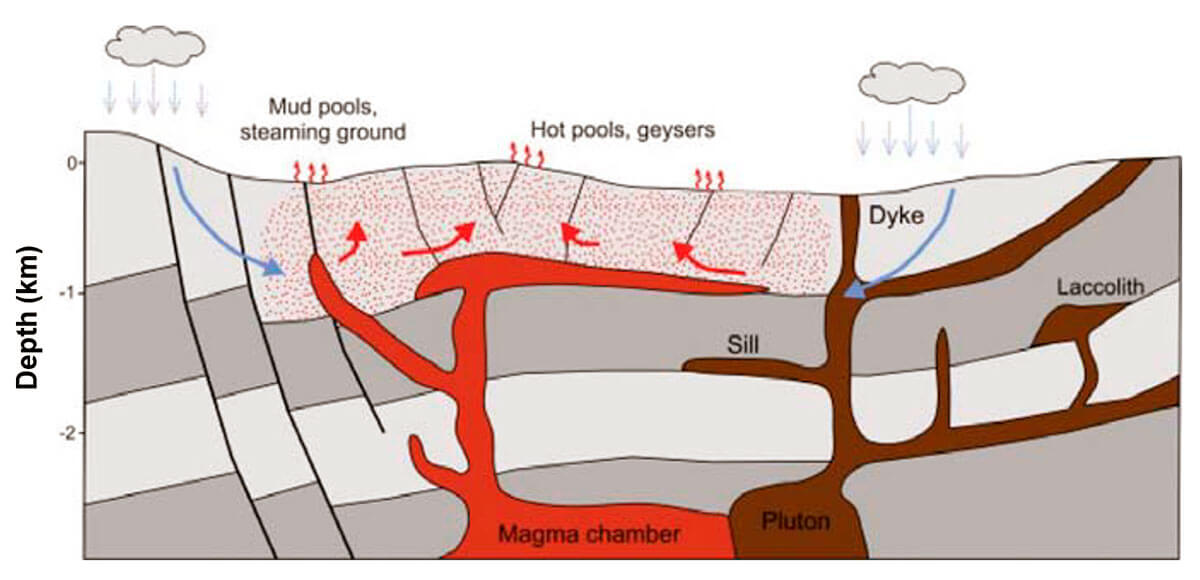
Additionally, other types of magmatic plays can be associated with extinct magmatic intrusions, where the heat source is a pluton or batholith, and surrounding hills or mountains provide a large recharge of circulating meteoric water that forms a hydrothermal system. Two of the largest geothermal fields in the world, The Geysers in California and Larderello in Italy, are both associated with large extinct magmatic intrusions.
Magmatic plays commonly show surface manifestations of an underlying geothermal system. Fumaroles, hot springs, mud pools, and geysers are all indicators of a possible high-temperature geothermal system. The flow of gases and hot water inside these geothermal systems are controlled by small fractures and faults in the volcanic rocks, where surface indications of an underlying geothermal system are observed along the fault planes at the surface.
Development projects over magmatic geothermal systems usually exploit a high-temperature reservoir specifically for power generation or combined heat and power. Power projects will typically utilize steam that comes out of wellbores (as either dry steam or flash steam separated from the liquid phase) (Figure 3). Electricity generation with geothermal steam is the most economical form of geothermal power generation, and the abundance of systems of this type close to plate boundaries make magmatic-based projects the most commonly developed.
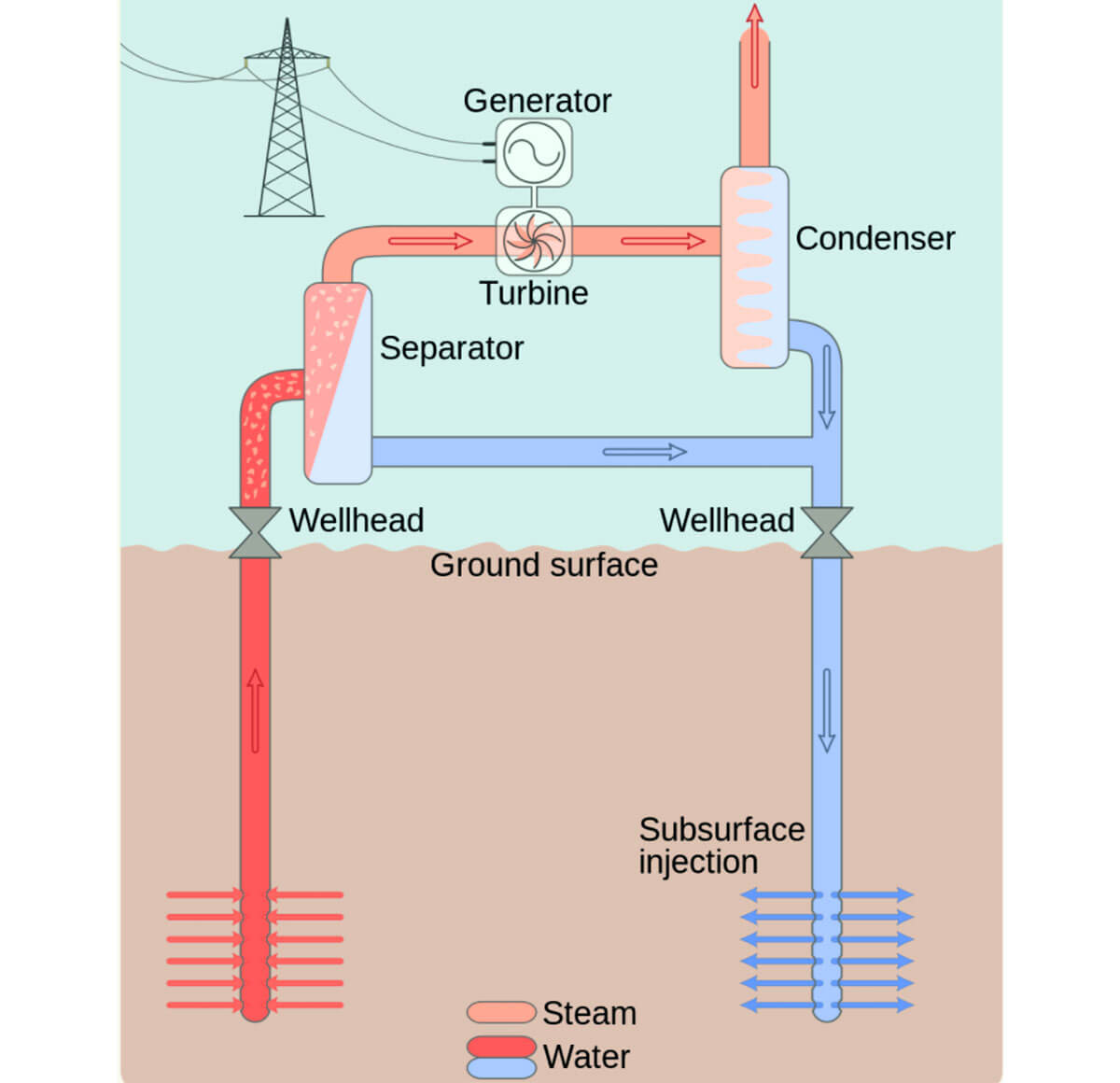
Extensional Domain Systems
Extensional domain geothermal systems differ from magmatic systems in that the heat source is created by an elevated mantle rather than a magmatic intrusive body. The elevated mantle is caused by crustal extension and thinning, which result in a high thermal gradient that heats meteoric water that infiltrates through shallow faults and circulates through deep faults and permeable formations. These systems tend to be fault-controlled (i.e., fluid movement and convection is controlled by faults and fractures produced by the local stress regime), as shown in Figure 4.
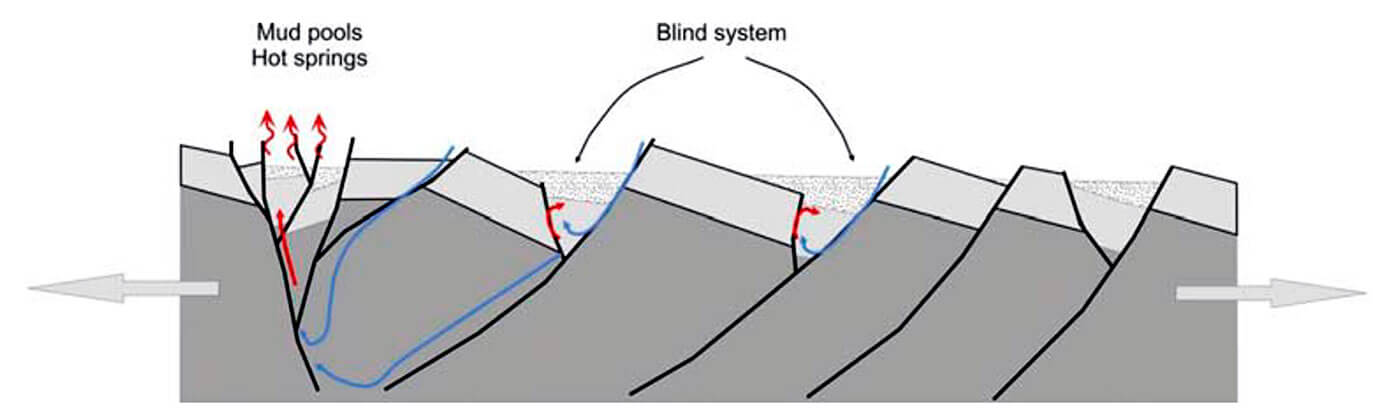
Extensional domain-type geothermal systems can be structurally complex and may or may not exhibit surface features (e.g., fumaroles, hot springs). The exploration effort for these systems consists of defining the local stress regime and the location of faults to identify the permeable faults and formations that may contain fluids. These types of systems are found in the Western United States, Turkey, and the East African Rift Valley, among other locations.
Depending on the temperature gradient, these types of resources may produce flash steam or hot water. The flash steam may be used to produce electricity directly, similar to the high-temperature magmatic systems, whereas hot water systems are used with a binary cycle to produce electricity (Figure 5). In a binary cycle, the hot water that flows out of the ground heats up and vaporizes a secondary fluid with a lower boiling point (the so-called working fluid), such as isopentane or isobutane, whose expansion into the gas phase drives the turbine. Note that the working fluid is not consumed in the process but is cyclically vapourized and condensed through heat exchangers in a closed loop.
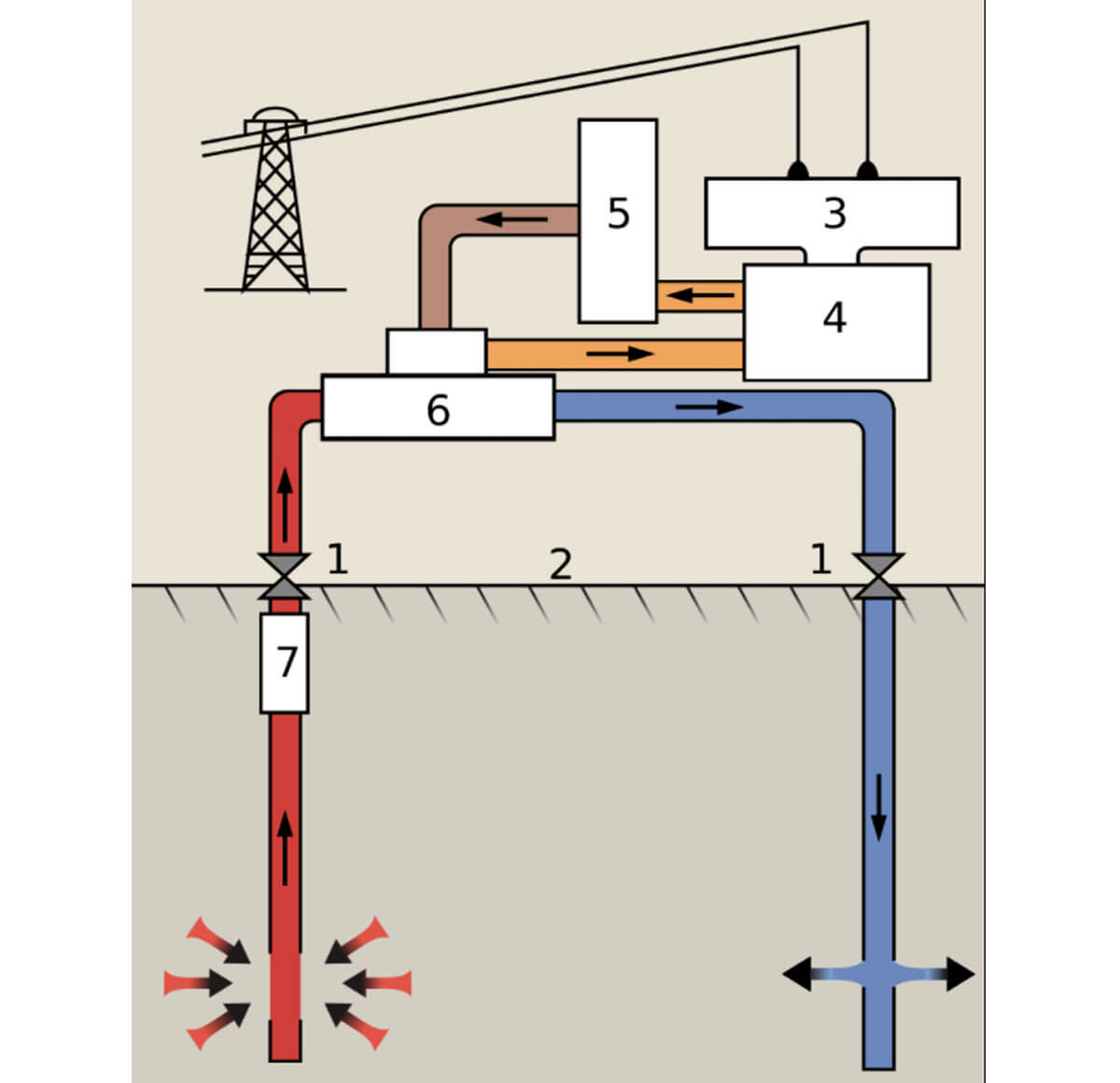
Conduction-Dominated Systems
The third category of geothermal systems is conduction-dominated systems, where fluid has been trapped and heated over time and there is no short-term convective flow. IGA (2014) divides these plays into three subcategories: (i) intracratonic play type, such as the North German Basin; (ii) orogenic belt type, such as in the Western Canadian Sedimentary Basin (Figure 6); (iii) basement type, as in the crystalline basement underlying thermally insulating sediments in the Big Lake Suite Granodiorite beneath the Cooper Basin in Australia.
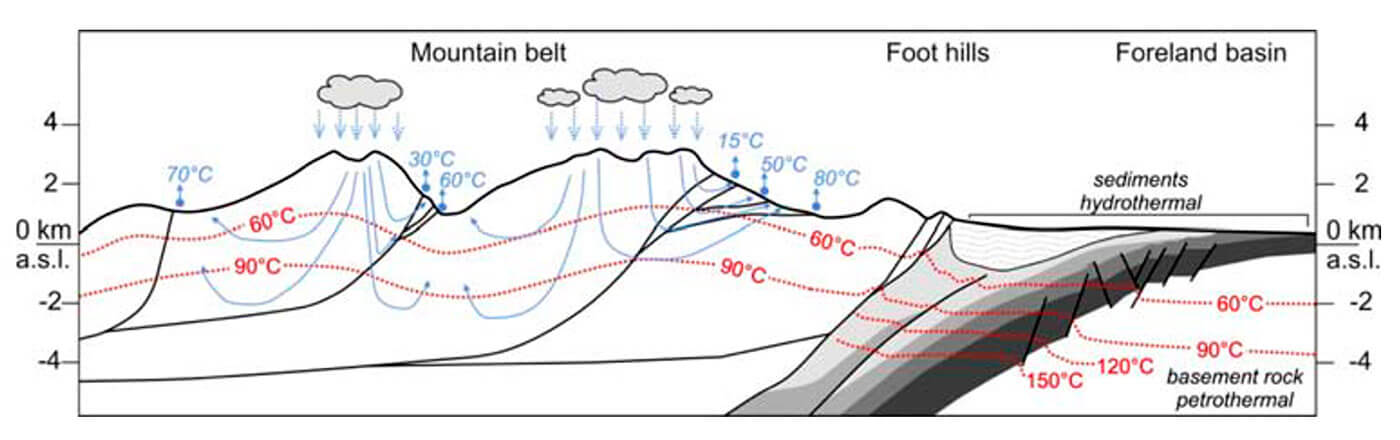
Conduction-dominated systems are not fault controlled, as there is little or no fluid movement, but faults may create barriers that trap a deep aquifer possibly in combination with tight sedimentary rock layers that overlay the aquifer as cap rock.
Because their locations are typically far from magmatic intrusions, the thermal gradient in conduction-dominated systems is less than in magmatic systems; therefore, the temperature of the resource is also lower. Power generation from these resources is done using binary cycle technology and typically relies on other direct uses of the heat (snow melting, space heating, etc.) in combination with power generation to make projects economically viable.
Geophysical Signatures of High-Temperature Geothermal Systems
The geologic components of high-temperature geothermal systems show strong contrasts in electrical resistivity, generally observed at depths greater than 1000m. Therefore, magnetotelluric (MT) methods, EM resistivity techniques with a great depth of investigation of several thousands of meters, are well suited to explore magmatic and high-temperature extensional domain plays. MT surveys are usually designed to cover a large area encompassing the heat source, the fluid upflow zone, the cap rock layer, and the outflow.
The typical conceptual model used for geothermal exploration over magmatic systems is shown in Figure 7. In this simplified model, fluids are heated by the magmatic intrusive zone, which in turn become buoyant and rise to the surface. The hot fluids drop in temperature as they rise, and through alteration form a smectite and illite-smectite layer that act as a low-permeability cap that prevents the buoyant fluid from escaping to the surface. The hot water will flow under this clay cap for some distance in what is called the outflow of the system.
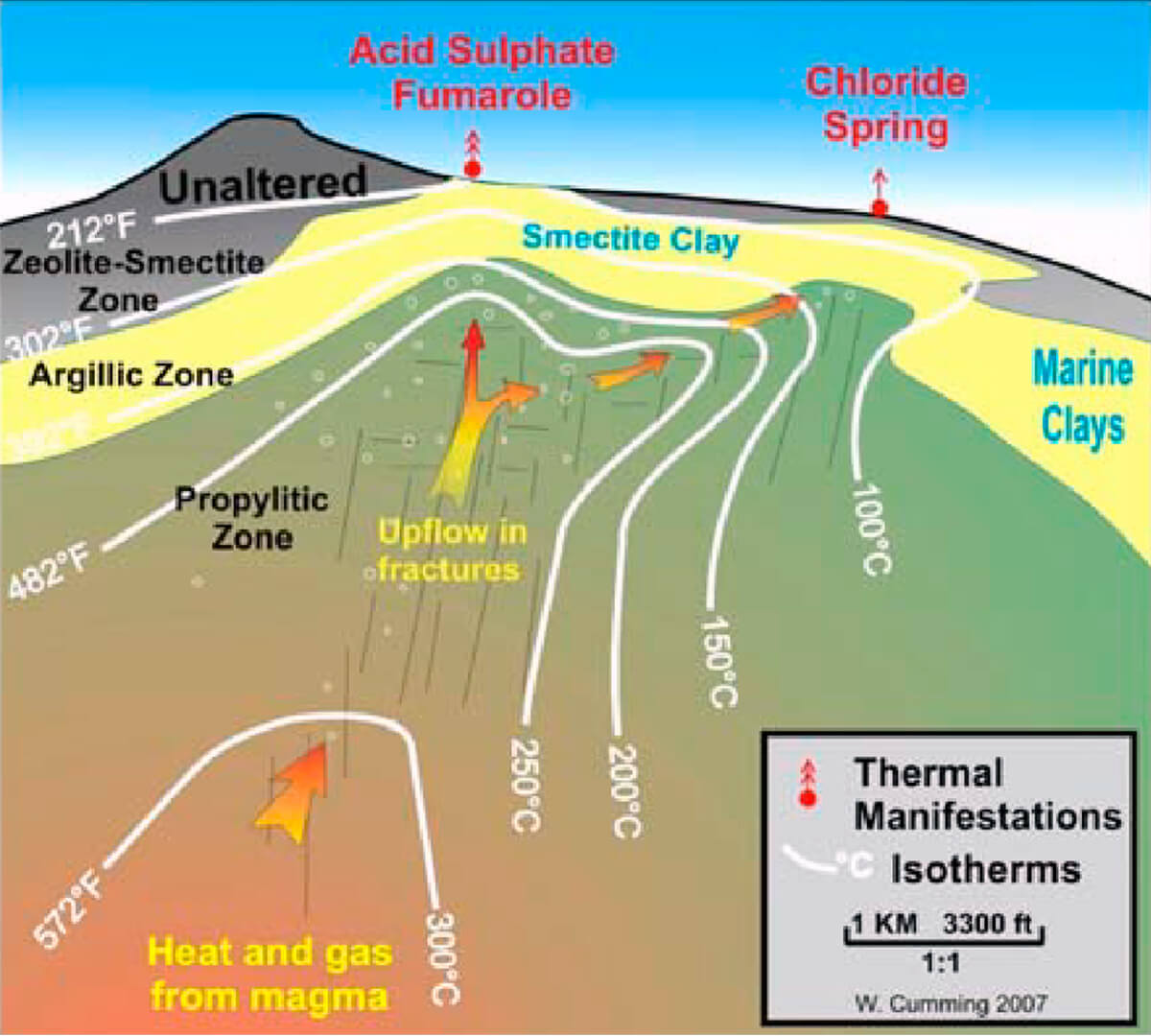
Commonly, there are three kinds of surface manifestations associated with high-temperature geothermal systems related to magmatic plays. Directly over the heat source acid sulfur springs and fumaroles with high gas content are generally observed, while over the upflow zone there will be acid sulphate fumaroles and Cl-SO4 pools. Further out in the outflow zone, where the fluid has depressurized and degassed, chloride springs with low gas content are more common.
However, lower-temperature systems, like most conduction-dominated plays, do not have the high temperature levels or acidic fluids that cause the formation of a clay cap, and therefore, the resistivity contrasts are not as pronounced. Integration of different geophysical methods are more commonly used to identify deep structures with low resistivity contrasts.
Typical MT Surveys for Geothermal Systems Exploration
Full tensor MT data is usually acquired over several sites across the geothermal system. The 2×2 data tensor over several decades of frequency bands represents resistivity variations in two horizontal directions as well as the vertical direction (i.e., the MT data is inherently three-dimensional). The data are later inverted to create 1D, 2D, and 3D resistivity distribution models over the survey area beyond the depth of interest. The 1D and 2D models are used during the data acquisition stage both to steer the MT site location and for quality control of the data as it is acquired. However, in order to adequately delineate complex systems, normally a full 3D MT inversion is conducted to produce the final interpretation model.
The typical MT signature of a high temperature geothermal system is a very low resistivity layer (< 10 Ωm) overlying a moderately low resistivity layer (10 to 60 Ωm). These resistivity responses are associated with the clay cap and the underlying propylitic reservoir with high chlorite and epidote content. The base of the clay cap layer is typically at 180-200°C, and the reservoir is at a higher temperature (Figure 8). These resistivity units, which are usually resolved at a close distance from a magmatic heat source, are typically overlain by a pattern of surface fumaroles and hot springs of varying chemical content and form the basic conceptual model for a high-temperature magmatic resource
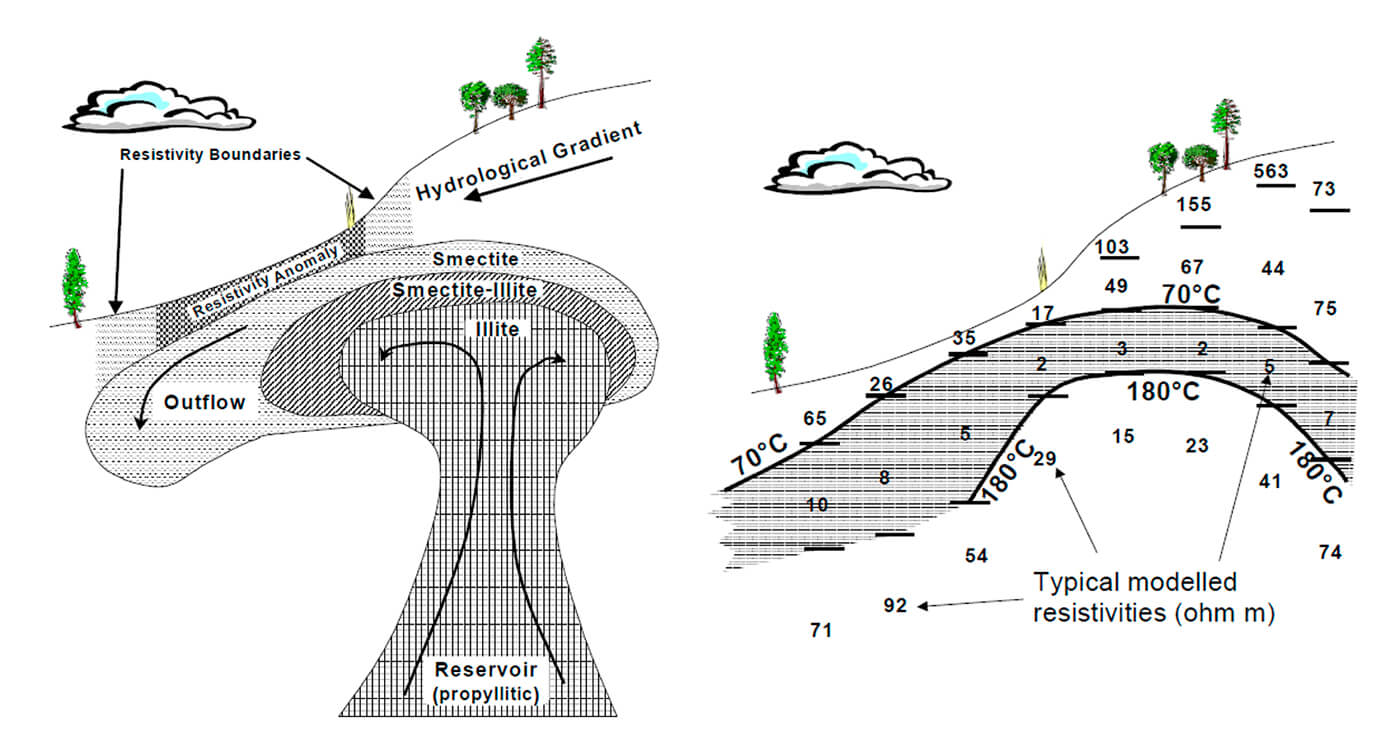
Figure 9 shows an example of a 3D MT model over a cross section of the Krýsuvík high-temperature geothermal area in Iceland (Hersir et al, 2013). The clay cap layer appears as low resistivity values of less than 10 Ω-m, while the underlying propylitic reservoir appears with somewhat higher resistivity values of around 100 Ω-m. The high resistivity near the surface corresponds to unaltered rock. Also, the MT resistivity model displays a close correlation with the borehole information.
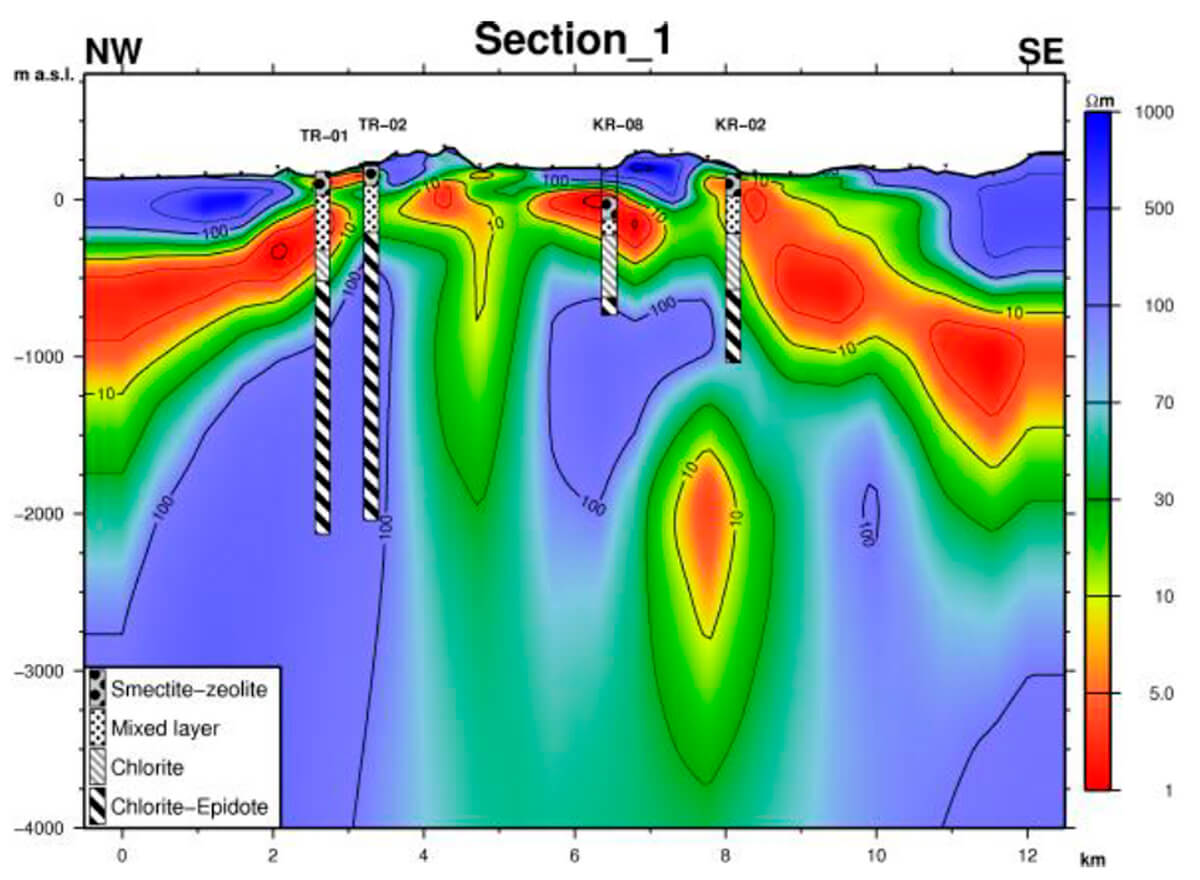
Another example of a magnetotelluric survey over a high-temperature geothermal system is given by Gharibi, et al. (2019). In this study, MT data was collected over the north side of the Fantale volcano in the East African Rift Valley in Ethiopia. As a first insight into the subsurface, the 1D models for the data show a low-resistivity zone at ~1400m depth, which would be a depth of economic interest (Figure 10).
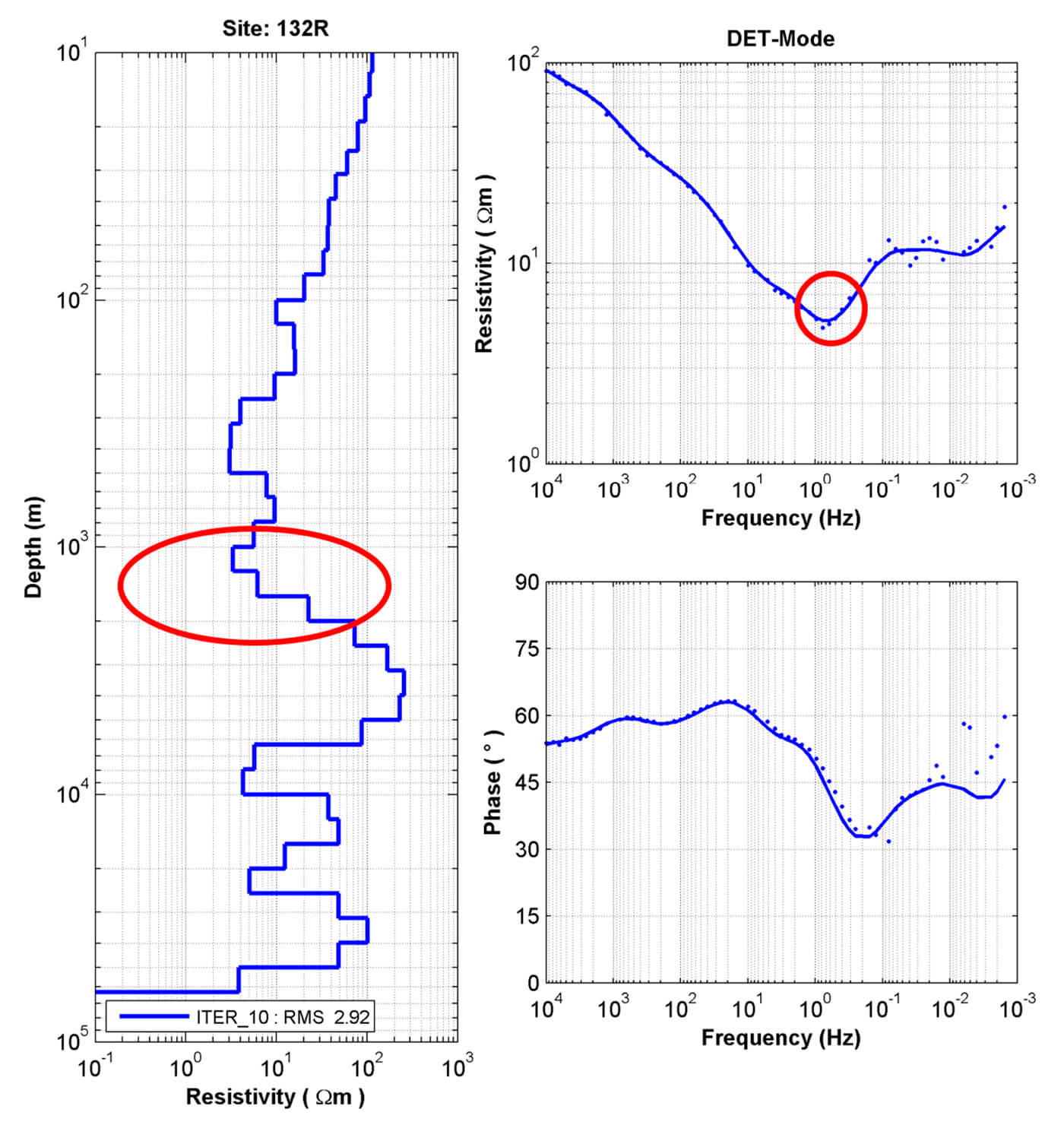
The low-resistivity zones can also be qualitatively investigated using the so-called induction vector, which is calculated using tipper components of the MT data. The tipper is the transfer function (i.e., ratio) between vertical and horizontal magnetic field at each MT site. A derivative of the tipper data, induction vectors are presented as arrows that point towards the conductor, and therefore, are diagnostic of conductive zones in the subsurface. The plot of the induction vector at 100Hz in the Fantale survey area does not show a clear alignment, indicating that there is no uniform conductive zone at the skin depth corresponding to that frequency (Figure 11). Skin depth is a measure of depth of investigation of MT data at a given frequency. The plot of the induction vectors at 0.1Hz, however, does clearly identify a zone of elevated conductivity, which may correspond to a clay cap layer.
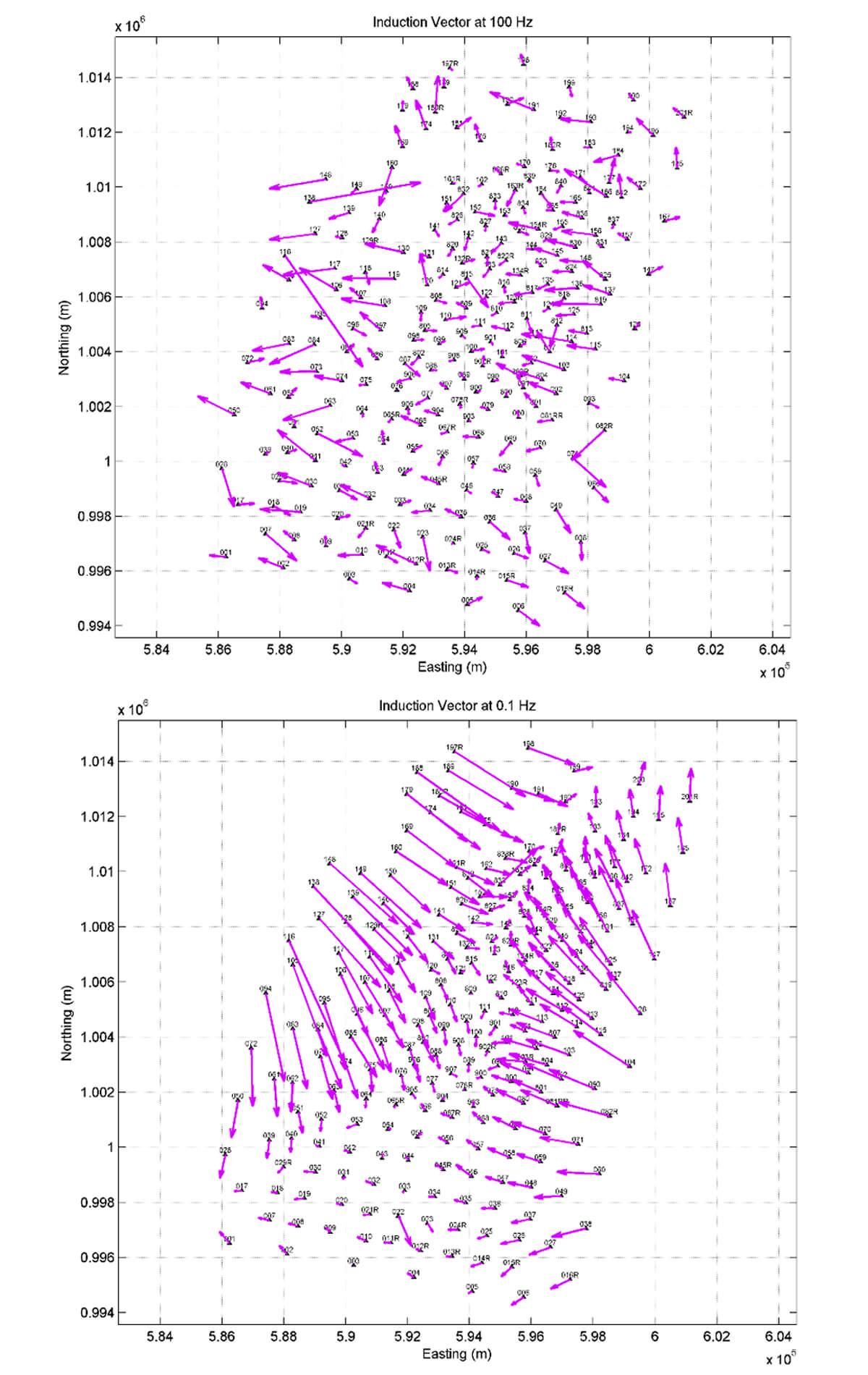
Additionally, the 3D resistivity model of the Fantale project identifies the potential heat source of the system as a column of low-resistivity material located immediately to the north of the crater, as observed in Figure 12.
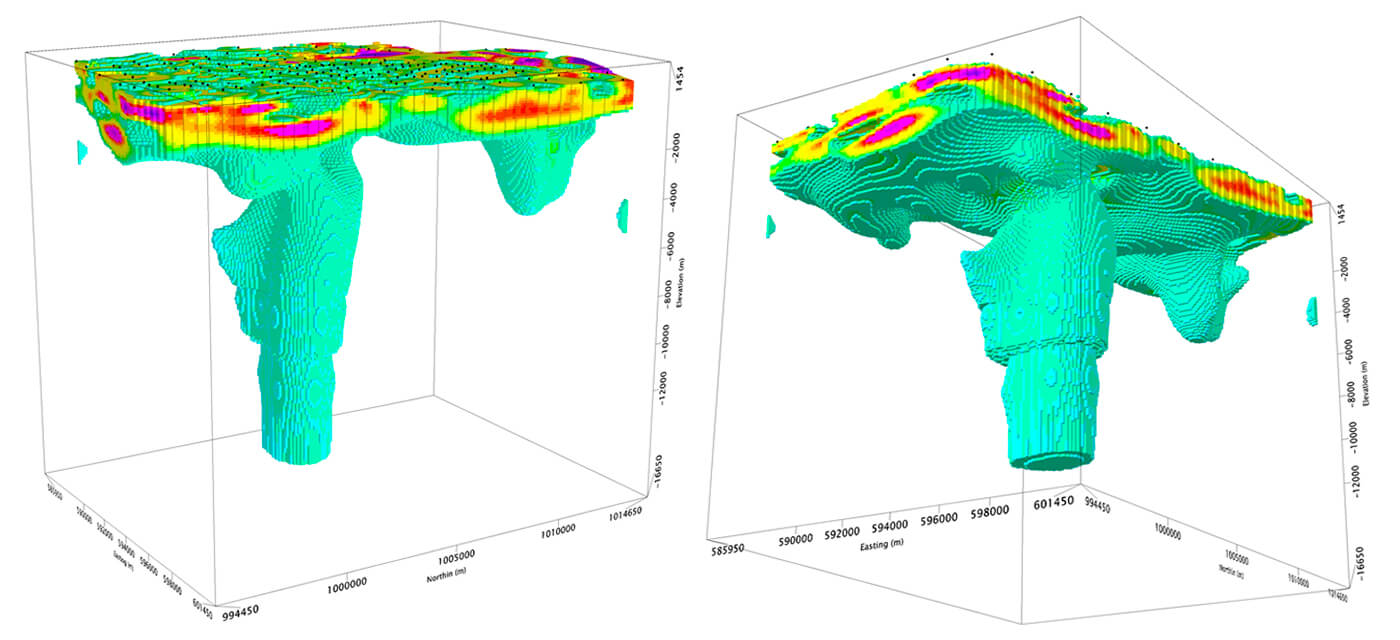
The constant-depth horizontal slices of the 3D model show a curving conductive layer at ~1600m depth overlying a slightly higher resistivity layer (but lower than the surroundings) at ~2000m depth (Figure 13).
The MT 3D resistivity image appears to show the classic upflow zone close to the heat source with outflow towards the north. This image, along with the location of fumaroles and hot springs, plus geothermometer data and surface geology observations, supports the existence of a high-temperature geothermal resource similar to the conventional conceptual model for these types of systems (Figure 7).
The resultant 3D model and constructed conceptual model specific to the system are then used to guide targeting and drilling. The shoe of the production casing should be located near the base of the interpreted cap rock layer in order to avoid circulation losses into the reservoir, which would also compromise the cementing of the production casing. The final stage of the exploration well is planned to penetrate the reservoir layer below the clay cap and should be lined with slotted or perforated liner. The hottest fluids are expected near the upflow zone, but also the content of non-condensable gases is expected to be higher, which is a consideration for the design of the power plant. In all these stages the MT model can be used to guide the design and the process.
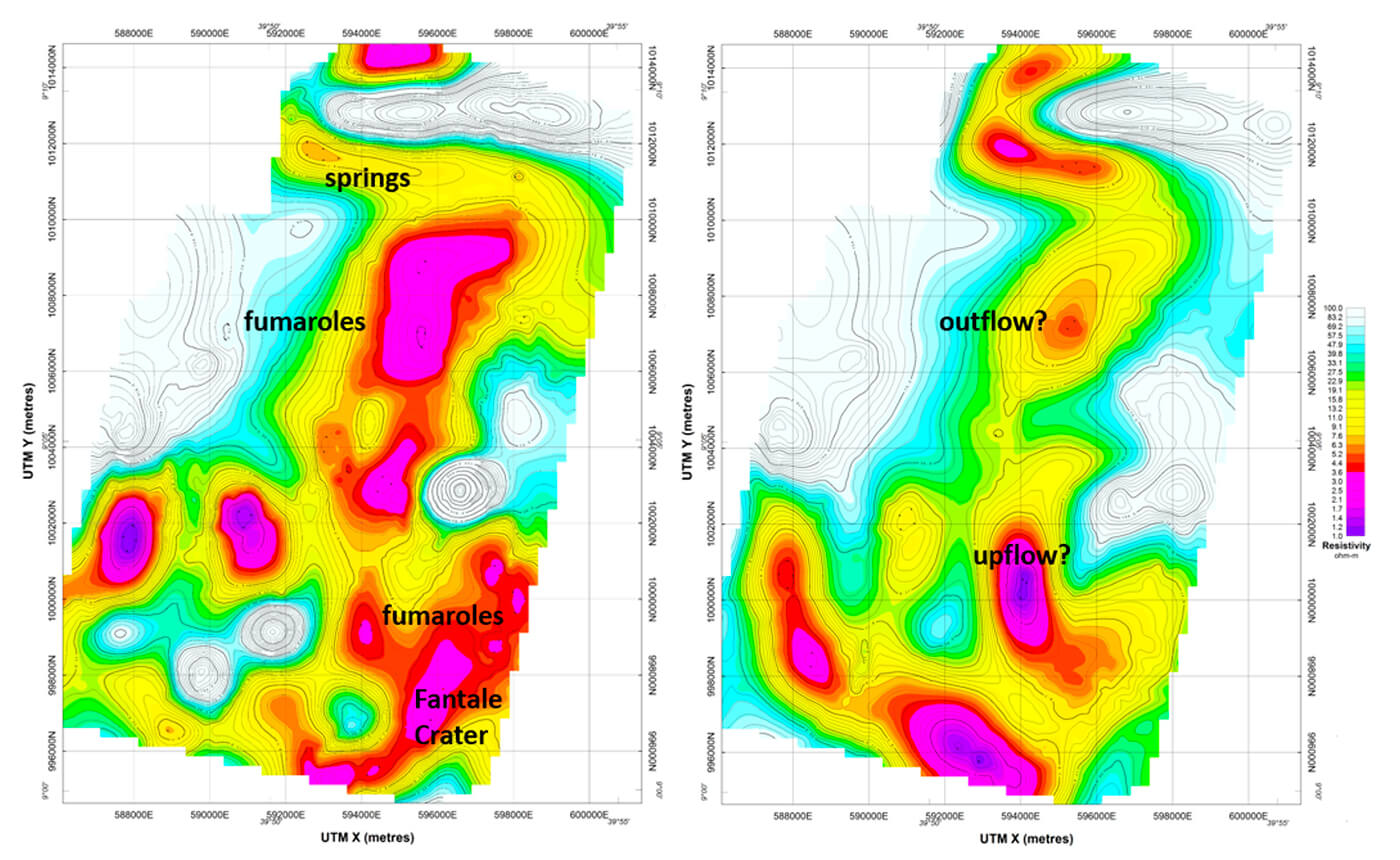
After exploration drilling is complete, the interpretation of the 3D MT model and the constructed conceptual model should be revisited and further refined by incorporating the information obtained from drilling and well logs. The revised conceptual model will lead to a numerical reservoir model that is instrumental in determining the choice of technology used for generation of electricity and/or other direct uses of geothermal heat.
Additionally, time-lapse magnetotelluric surveys can be conducted to monitor the state of a geothermal system during production and exploitation to extend the longevity of production and improve the economics.
Conclusions
High-temperature geothermal systems can be developed economically in many locations around the world for the generation of electricity, direct use of heat, or hybrid projects. The economics of geothermal projects are largely driven by the temperature and depth of the specific hydrothermal system, therefore development and understanding of the geoscientific data and models are critical in the assessment and feasibility stages and for a successful development of the geothermal resource.
Hydrothermal systems located close to volcanoes – active or extinct – or over areas of high thermal gradient form convective cells of fluid circulation that over time form an altered clay layer, with low electrical resistivity property, that acts as a cap rock layer over the geothermal reservoir. The components of these high-temperature geothermal systems: the heat source, the cap rock layer, structures that can control fluid flow, and the high-temperature fluid in a rock matrix, all produce resistivity signatures that can be captured and identified by magnetotelluric surveys.
The resulting magnetotelluric resistivity models, together with geochemical data from fumaroles and hot springs that form at the surface, and geologic and structural mapping are integrated to produce a conceptual model of the geothermal system. This conceptual model is used to guide target drilling and evaluate the economic viability of geothermal projects.
Given the large capital costs involved in development of industrial-scale geothermal projects, the value of MT surveys has been well established. When properly executed, MT surveys can de-risk geothermal exploration and exploitation and maximize the likelihood of successful technical and economic outcomes.
Join the Conversation
Interested in starting, or contributing to a conversation about an article or issue of the RECORDER? Join our CSEG LinkedIn Group.
Share This Article