Abstract
Despite the success of multi-component seismology and associated converted-wave (PS-wave) imaging methods in the petroleum industry, there has been relatively little effort devoted to shallow, high-resolution converted-wave imaging in the coal sector. By analogy to petroleum-scale applications, converted-wave seismic imaging in the coal environment offers interesting possibilities for independent validation of mapped structures, clearer imaging in the shallow subsurface, the detection of gas, and the extraction of lithological information away from borehole locations.
Over the past five years Velseis Pty Ltd has conducted the first experiments in Australia to utilise shallow, high-resolution multi-component seismic data and converted-wave technology to image coal seams. In total, seven 2D multi-component coal-seismic datasets have been acquired in the Bowen Basin, Australia. Only minimal changes to conventional recording equipment and procedures have been required. Conventional dynamite and mini-SOSIE sources have been used, with a single, multi-component geophone replacing the conventional array of geophones at each receiver station.
Converted-wave processing algorithms developed within the petroleum sector have been applied successfully to the 3C coal-seismic datasets. Considerable experimentation to fine tune processing parameters and the converted-wave processing sequence has been necessary for optimum handling of the shallow, high-resolution data. Overall, the processing of the PS-wave data has been significantly more challenging, and has required more geological input, than conventional P-wave processing.
This research has demonstrated that converted-wave imagery is viable in the shallow coal environment. Field trials have demonstrated that the converted-wave seismic image can be competitive for structural imaging, and a number of the field trials have demonstrated significant advantage in imaging very shallow coal seams with PS waves. Converted-wave trials in multi-seam environments have illustrated the potential of integrated P/PS imagery for extracting lithological information away from boreholes.
Introduction
Seismic reflection is a valuable geophysical tool for accurate and cost-effective imaging of coal seams and is of significant importance to the economics of coal mining in Australia. However, the desire to locate and characterise increasingly subtle structures and lithological features continues to drive research into alternative seismic technologies, including multi-component seismology.
Multi-component seismology has received considerable attention in the petroleum sector. Remarkable advances have been made in multi-component acquisition and processing in recent years and there are a number of examples where converted-wave seismic techniques have proven more successful than conventional seismic imaging methods (e.g., Barkved et al., 1999; Engelmark, 2001).
In contrast, there has been little effort devoted to shallow, high-resolution converted-wave imaging in the coal sector. This is despite the knowledge that coal seams generate particularly strong converted waves (e.g., Fertig and Müller, 1978; Greenhalgh et al., 1986). Converted-wave imaging in the coal environment has the potential to provide independent validation of mapped structures and clearer or more coherent sub-surface images. There is also potential for the detection of gas, sandstone channels and other lithological anomalies. With the support of the Australian Coal Association Research Program (ACARP) and local mining companies, Velseis Pty Ltd has conducted a number of experimental and commercial multi-component seismic trials in the Bowen Basin, Australia (Velseis, 2003; Hendrick, 2005). This paper summarises our key results to date. It demonstrates that converted-wave imagery is viable in the shallow coal environment and that it can extend the results achieved with conventional P-wave seismic reflection.
Converted-Wave Seismology
In brief, seismic reflection involves imaging the sub-surface using artificially-generated sound waves. Typically, small dynamite explosions or vibratory sources (eg, Mini-SOSIE; Vibroseis) are used to generate seismic waves for coal exploration. Surface receivers (geophones) are used to detect the seismic energy that originates from the seismic source, travels down into the earth and gets partially reflected back to the surface at geological boundaries.
The conventional approach to coal-seismic acquisition incorrectly assumes that typical coal-seismic sources will result in only compressional (P) waves arriving at the surface. P waves are longitudinal sound waves that have particle motion in the direction of travel. Hence, reflected P-wave energy travelling upwards from a geological boundary will have particle motion with a strong vertical component at the surface receiver (Figure 1a). Conventional seismic acquisition records only the vertical component of seismic energy arriving back at the receiver. This type of seismic recording can also be referred to as single-component recording.
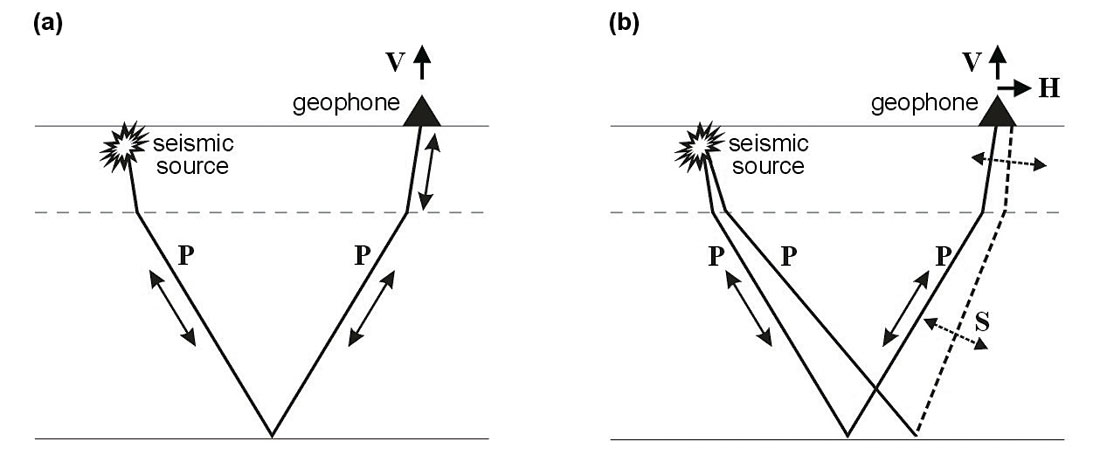
In reality, both reflected P and shear (S) waves typically arrive at the surface during a seismic survey. S waves are transverse sound waves that have particle motion perpendicular to the direction of travel. Since coal-seismic sources dominantly
produce P-wave energy, most of the S energy arriving at the surface is in fact mode-converted PS energy. That is, energy from a wave that travels down to a geological boundary as a P wave, gets partially converted to S energy at the boundary and then travels back to the surface as an S wave. Any PS-wave energy arriving at the surface will have a strong horizontal component of particle motion (Figure 1b). Multi-component seismic recording measures both the vertical and horizontal components of ground motion to enable exploitation of both the P and PS energy arriving at the surface. Note that multi-component recording may also be referred to as three-component (3C) recording, since the vertical and two orthogonal horizontal components (inline and crossline components) of ground motion are generally recorded.
The different modes of propagation of P and S waves mean they travel at different speeds through the earth and respond differently to various geological situations. For example, S waves typically travel at about half the speed of P waves. This is particularly relevant to the imaging of very shallow coal seams (less than approximately 50 m deep). PS waves reflected from very shallow coal seams will generally arrive after near-surface noise that contaminates the corresponding P-wave reflection energy. In addition, P waves are influenced by pore space and/or fluid and gas saturation, while S waves are not. This is because S waves will only travel in solid materials. This difference in behaviour makes integrated use of P- and S-wave information potentially valuable for helping better understand lateral variations in sub-surface lithology.
Multi-Component Acquisition
Converted-wave seismology utilises an efficient acquisition strategy which can accommodate both P-wave and PS-wave recording through use of a conventional P-wave seismic source. To date seven 2D-3C field trials have been carried out at coal mines in the Bowen Basin, Australia. These data have been acquired using both buried dynamite and surface mini-SOSIE sources and only minimal changes to conventional recording equipment and procedures have been required to take advantage of PS energy arriving at the surface. The primary difference is the receiver device. A purpose-built high-resolution, high-output 3C geophone replaces the array of vertical geophones used for conventional acquisition at each receiver station. Each 3C geophone is levelled and aligned for acquisition manually. Note that arrays of 3C geophones are not used since S waves are very sensitive to lateral variations in the near surface, and geophone arrays would severely distort any S energy arriving at the surface (eg, Hoffe et al., 2002). Our field experiments indicate that single-geophone acquisition does not compromise the quality of the conventional P-wave data.
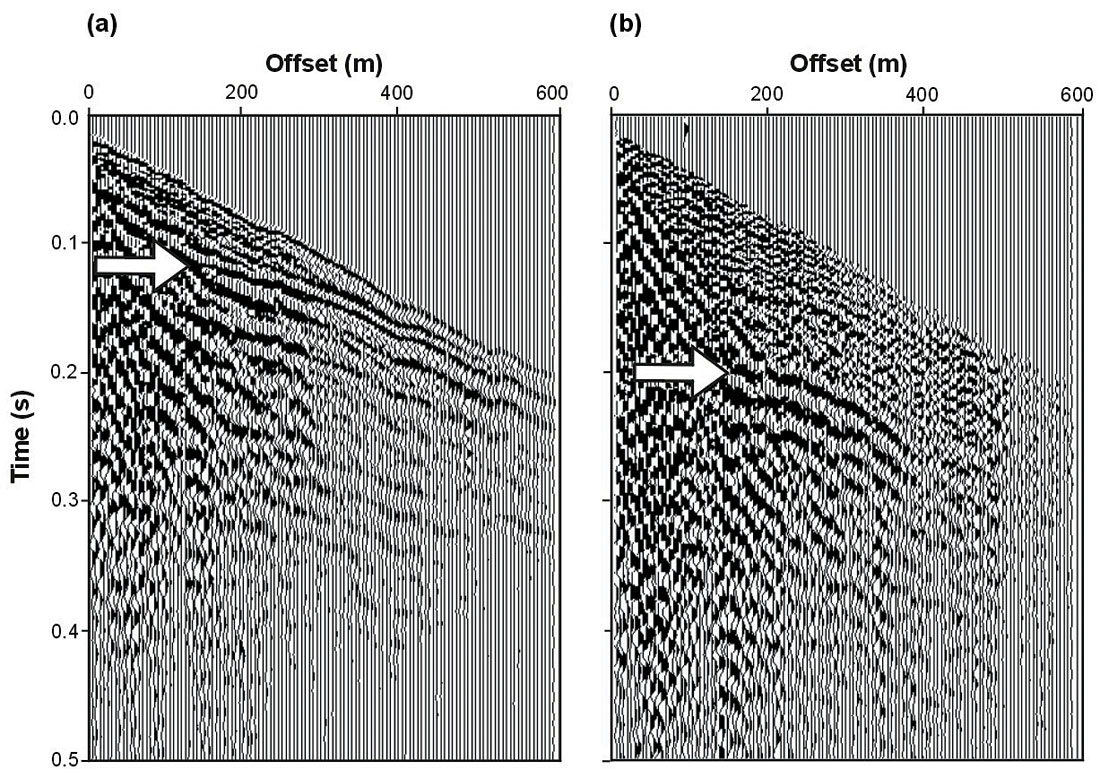
Figure 2 shows a representative multi-component seismic record (vertical and inline components only) from Field Trial A. These data have been acquired using a buried dynamite source in an area that has a single, thick coal seam at relatively shallow depths. On the vertical component the P-wave reflection from the target coal seam is at 0.1 s at zero offset. The strong PS reflection event on the inline component (0.2 s – 0.25 s on centre traces) demonstrates strong conversion is occurring at the target coal seam. To date, no significant PS energy has been identified on the crossline components of data. Figure 3 shows a representative multi-component seismic record from Field Trial B. These data have been acquired using a surface mini-SOSIE source in an area of multiple, shallow coal seams. On the vertical component, P-wave reflection energy can be identified at 0.1 s at zero offset. Strong PS reflection energy can be seen at approximately 0.13 s on the inline record. Note that, in contrast to the dynamite data in Figure 2, the data in Figure 3 suffer from strong surface-wave contamination and SS refractions and reflections related to the surface seismic source. Note also that the inline records in Figures 2 and 3 are noisier than the corresponding vertical records. This is typical of all of our multi-component datasets. As a consequence, the final PS images suffer from poorer signal-to-noise ratio than the conventional P-wave images.
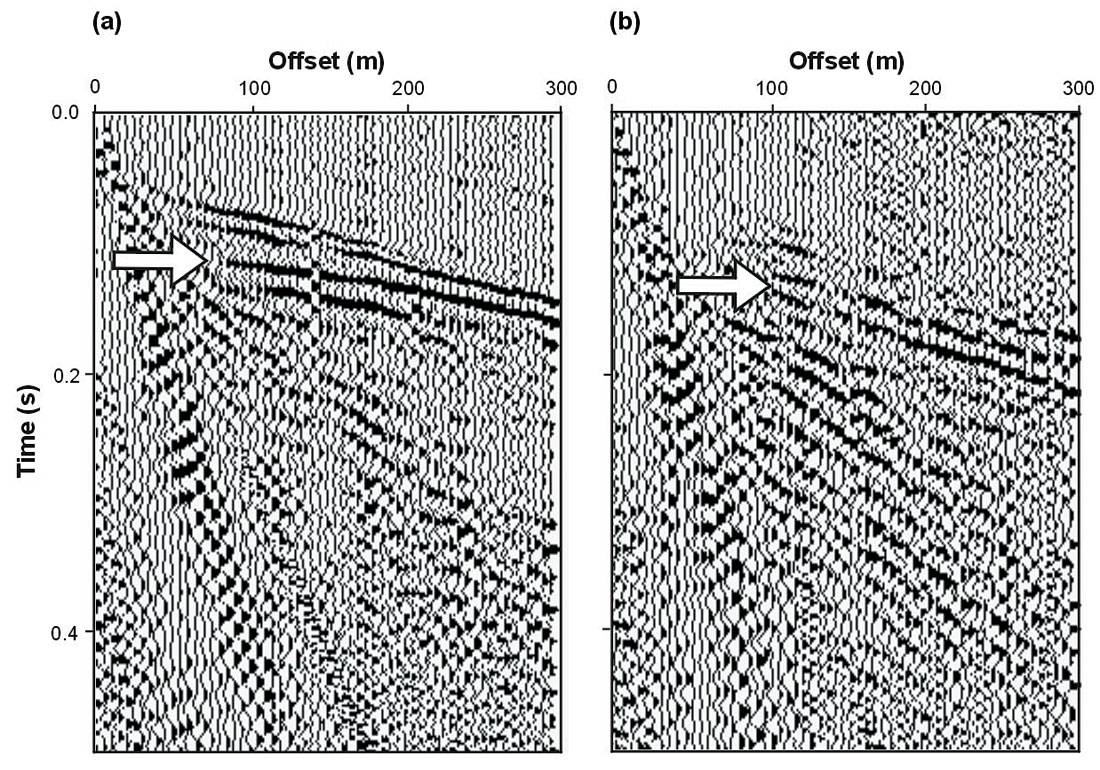
Converted-Wave Processing
Processing of converted-wave seismic data involves a number of steps that are substantially different from, and significantly more challenging than, conventional P-wave processing. In particular, converted-wave processing involves specialised approaches to S-wave receiver statics, PS normal moveout (NMO) correction and common conversion-point (CCP) binning.
Perhaps the most critical stage of processing high-resolution converted-wave data involves the computation of S-wave receiver statics. This information is required to compensate for delays associated with converted S-wave energy travelling through the weathered layer. Our initial attempts to compute S-wave receiver statics utilised the automatic common-receiver-gather (CRG) stack-power optimisation method of Cary and Eaton (1993). However, their assumption that very little structure should affect the PS reflection event used to define the static corrections was too limiting for our high-resolution coal-seismic data. Instead, a new S-wave statics method has been developed, whereby the traveltimes of a specific PS reflection event on each common-offset receiver section are used to solve for the S receiver statics in the surface-consistent statics model in a least-squares sense.
PS reflection events exhibit non-hyperbolic NMO which is dependent on Vp, Vs, the depth of the reflector and the horizontal distance from the source point at which the conversion occurs (e.g., Zhang, 1996). P-wave velocities (Vp) are extracted from conventional P-wave processing of the vertical component of data. Velocity analysis for converted-wave data is designed to recover S-wave velocities (Vs), or equivalently the P-wave to S-wave velocity ratio (Vp/Vs). Our preferred velocity analysis tool produces a suite of constant Vp/Vs stacks and involves rebinning the data for each trial velocity ratio. (This approach is analogous to using conventional constant-velocity stacks.) We have found it necessary to handle positive and negative offsets independently so as to work around the diodic (ie, non-symmetric) moveout observed in CCP gathers.
The raypath of a PS wave through the Earth is asymmetric. This is because the upcoming S wave travels more steeply than the downgoing P wave. Consequently, the reflection point of a PS wave is offset from the midpoint of the source and receiver towards the receiver. The precise location of this reflection point is actually a function of Vp/Vs, and will vary with the depth of the reflector. The process of forming common conversion point gathers is referred to as CCP binning (e.g., Zhang, 1996). Practical experience has demonstrated that ‘dynamic’ CCP binning yields the best results for shallow, high-resolution converted-wave imaging. This approach bins each sample down each trace according to its two-way time and the local Vp/Vs value. Thus portions of seismic traces can be assigned to different CCP bin locations.
Interpretive Results
Processing of our converted-wave data has proven non-trivial. Nevertheless, viable PS images have been achieved for all of our trial multi-component surveys. Here I comment on some of the more interesting interpretive results.
The final P and PS images for a portion of one of the 2D lines acquired from Field Trial B are shown in Figure 4. As is conventional practice, the vertical scale of the PS image has been adjusted (based on an estimated P-wave to S-wave velocity ratio) to provide a comparable depth perspective to the P-wave image. The PS image is competitive for structural imaging, providing independent validation of the major structural features.
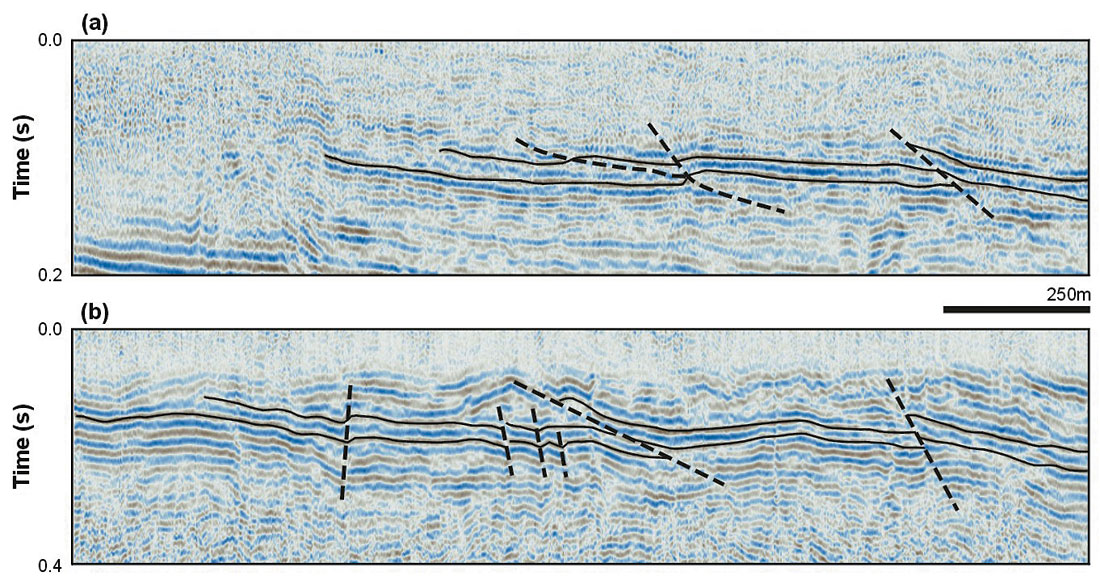
However, the most significant outcome of this experiment has been the success of the PS data to image very shallow coal seams across the left end of the line. In contrast to the target P-wave reflection energy, the later-arriving PS energy has not been eliminated by near-surface noise. Consequently, the PS data can effectively map the target coal seams across the full extent of the survey line, to depths of 25–30m. The PS interpretation results along the left hand end of this line have been tested with borehole drilling, and have been proven accurate. The outcomes from this experiment, and another earlier trial designed to image a shallow coal seam, have resulted in a number of multi-component seismic surveys specifically targeting open-cut coal reserves in the Bowen Basin, Australia.
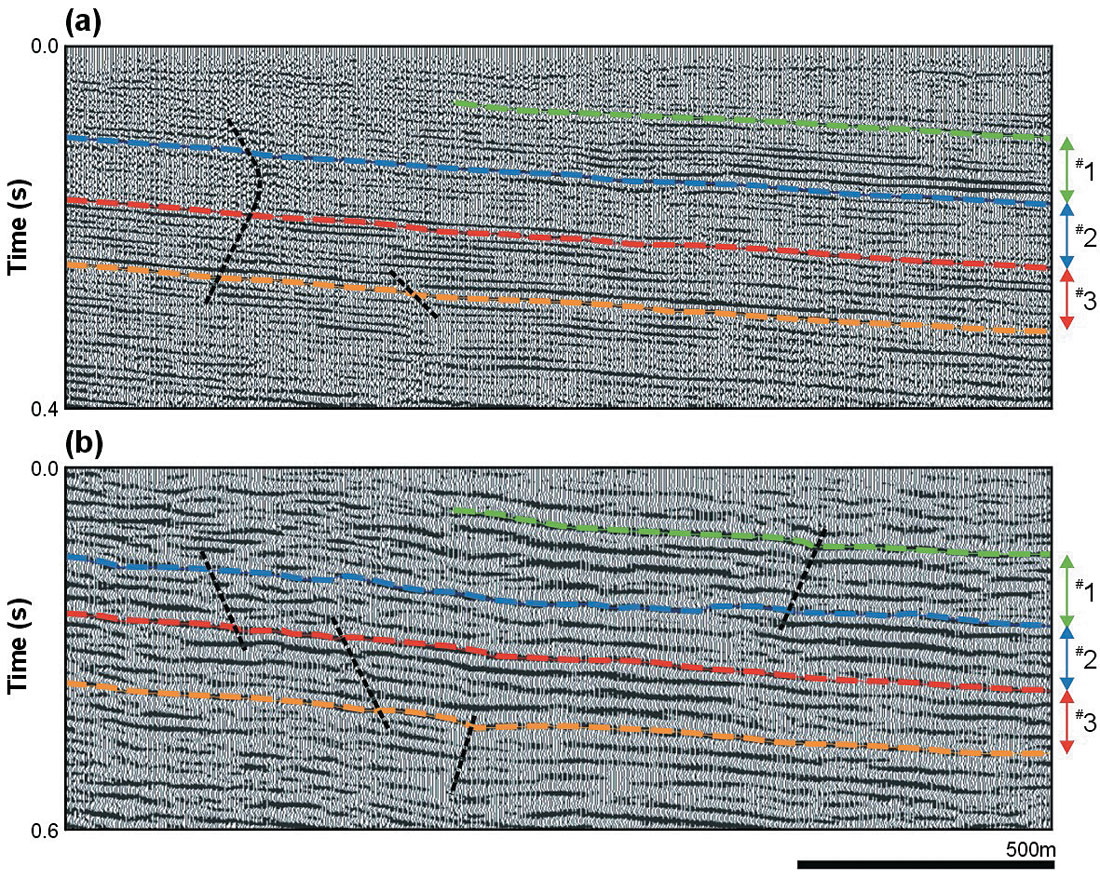
Figure 5 shows the final P and PS images from Field Trial C, which was an experiment conducted using a buried dynamite source in a multi-seam environment. It is believed a thick, poorly consolidated near-surface layer has resulted in significant attenuation of upcoming S energy. Consequently the PS image has reduced resolution compared to the P-wave image and does not add significantly to the structural interpretation. However, this multi-seam example does illustrate the potential of using integrated P/PS imagery for extracting lithological information away from boreholes. In Figure 5 significant geological interfaces have been identified on both the P and PS images. Corresponding time intervals have been measured to yield interval P-wave to S-wave velocity ratios (Vp/Vs). Figure 6 shows the lateral variation in Vp/Vs for the three geological intervals of interest. The uppermost interval (small dash - green) exhibits generally high Vp/Vs ratios, as might be expected in the shallower, less-consolidated part of the section. The second interval (dash - blue) exhibits an abrupt lateral change in Vp/Vs (at distance of approximately 1200 m). This is consistent with a change from shale-rich material on the right to sandy (or possibly gas-contaminated) material on the left. The deepest interval (solid - red) lies immediately above the target coal seam. This exhibits a more consistent Vp/Vs ratio, indicative of a shale-rich sequence. The abrupt increase in Vp/Vs at a distance of 500 m for Interval 2 and at a distance of 750 m for Interval 3 may be indicative of unconsolidated material associated with fracturing about a small fault. Note that geological information from boreholes has yet to become available for confirmation of any aspects of this interpretation. An identical experiment along a 2D line with a large amount of borehole control is currently being undertaken to test the relevance of this type of analysis for coal environments.
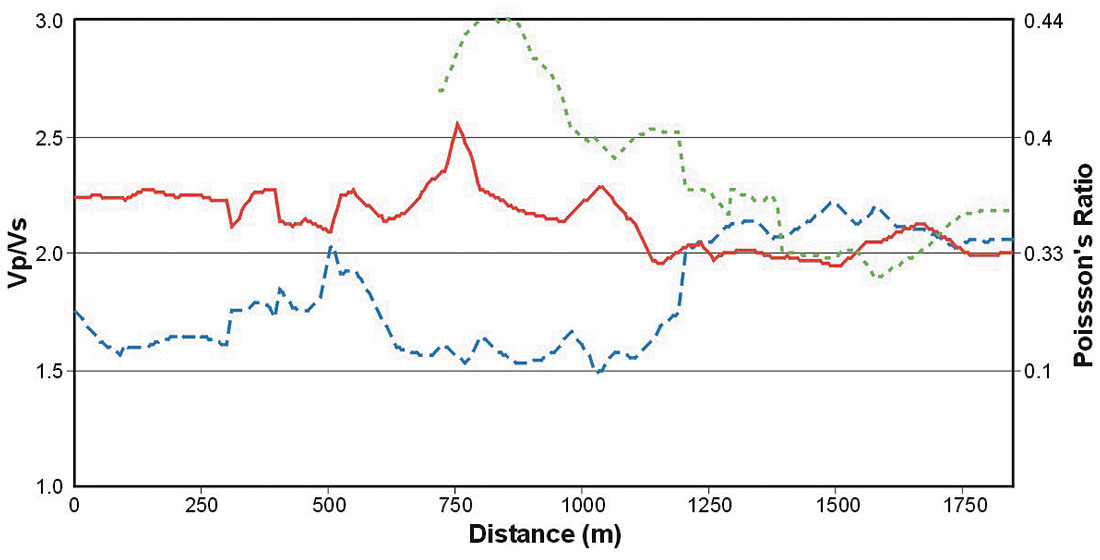
Conclusion
Velseis Pty Ltd has undertaken the first evaluation of multi-component seismic acquisition and converted-wave technology in the Australian coal environment. Combined P/PS datasets have been recorded with minimal adjustment to standard P-wave acquisition procedures. Processing of our converted-wave data has proven challenging, particularly in the definition of severe S-wave receiver statics. Nevertheless, viable PS images have been obtained. In general, our research has focused on three objectives – to examine the potential of converted-wave data to yield (i) higher resolution images of the sub-surface at typical coal depths, (ii) viable images at very shallow target depths and (iii) lithological information away from borehole locations. In the majority of the field experiments, the PS image has proven competitive for structural imaging, providing independent validation of features. However, the resolution of our PS images has, at best, only been comparable to that of the conventional P-wave images. Two of the trials have demonstrated a significant advantage in imaging very shallow coal seams with PS waves. This has resulted in a number of new multi-component surveys targeting open-cut coal reserves. In addition, our converted-wave trial in a multi-seam environment has illustrated the potential of integrated P/PS imagery for mapping lithological variations away from boreholes. Hence, our results to date suggest that achieving the latter two objectives routinely with shallow, high-resolution converted-wave seismic may be realistic. As the technology is refined, the extra costs associated with the acquisition and processing of converted-wave data could be justified in terms of increased information content.
Acknowledgements
This research is receiving ongoing financial support from the Australian Coal Association Research Program (ACARP Projects C10020 and C13029). The author would like to thank the Bowen Basin host mines for their support of this research. Thanks also to Helen Richmond (Senior Technical Officer, Velseis) for her assistance with preparing the figures for this publication. The use of Seismic Un*x from CWP, Colorado School of Mines is acknowledged as our seismic software development framework.
Join the Conversation
Interested in starting, or contributing to a conversation about an article or issue of the RECORDER? Join our CSEG LinkedIn Group.
Share This Article