Abstract
Natural gas hydrates are crystalline structures that combine natural gases and water under appropriate temperature and pressure conditions. Natural gases and water are found throughout the Solar System, even in interplanetary space. It is geologically reasonable to conjecture that these natural components were incorporated into the proto-Earth and that hydrates formed as soon as the appropriate temperature and pressure conditions evolved. On Earth today, a planet long dominated by plate tectonics, hydrates of methane and other gases, hydrocarbons, carbon dioxide, and hydrogen sulfide, are found across polar zones and in many marine sediments. It is almost certain that hydrates have existed at least as long as have plate tectonics, with these tectonics creating geologic circumstances similar to those at present although distributed differently over the Earth’s surface.
Petroleum exploration to ever-greater water depths as well as greater depths into the subsurface is continuing to reveal that continental margins, both active and passive, are surprisingly complex, dynamic, and often surprisingly structurally weak. Numerous processes interrelate on scales from the minute to the regional and in intricate manners. The complexity of features observed along margins, by tools that observe the small to the large, from bottom photographs and cores to industrial multichannel seismic reflection records, respectively, implies a diverse geology and stress regimes. These interrelationships are illustrated along a dip section that trends from northwest to southeast and starts at the continental shelf break, crosses the upper continental slope and a mid-slope basin and proceeds down the western flank of the pronounced Mississippi Canyon along the northern Gulf of Mexico continental margin. The boundaries of the mid-slope basin are defined by two diapiric salt structures. This specific description is made to facilitate understanding of the geologic complexities, including a role for hydrates that appear to be common along many continental margins.
Numerous near-seafloor faulted blocks are observed on boomer, chirp sonar, and G.I.gun profiles along the slope-descending seismic data. These data also show the sediments near the sea floor to be characterized by weak, yet interpretable, reflectors. The weak reflectivity may indicate cementation by gas hydrates. In places, the weak reflectors are underlain by chaotic, sharply stronger reflectivity typical of free gas.
The boomer and chirp profiles over the mid-slope basin exhibit vertically oriented structures within the near-seafloor sediments, which may mark pathways of small-scale fluid expulsion, the fluid possibly being produced by dissociation of gas hydrates. The pathways may coincide with sites of weakness produced by gravity-induced tension. Also, mound-shaped features occur on the sea floor within the mid-slope basin. One of these has been documented to be a mud diapir containing gas hydrates.
Heat-flow measurements over the mid-slope basin indicate that the sea floor there is within the hydrate stability zone and that the zone is some 500m thick. Heat flow at the base of the hydrate-bearing diapir is 10-fold greater than that at its summit. Combining the high heat flow with seismic evidence that the base of the diapir comprises a fault scarp leads to an interpretation that warm fluids are flowing from an “open” fault there.
The tectonic matrix is complex and dynamic. Overall area motions are gravity-driven subsidence with evidence of rising tectonics: local thermal gradients, buoyant salt, mud diapirism, and fluid expulsion. The shallow and regional geology thus provide detailed input for ever-more-quantifiable margin-spanning models.
Two principal ideas are put forth; 1) that hydrates appear to be features whose existence and permanence are really ancient and 2) that a general description of contemporary (uppermost Holocene) hydrate occurrence within the geologic complexity of the northern Gulf of Mexico can be made from analyzing recently-collected data. Previous high-stand events presumably created similar geologic situations: the present may be a key to the past.
Introduction
Central to current knowledge of the seafloor and sub-seafloor, is the contribution of remotely acquired seismic data. With an oceangoing vessel and a system capable of dispensing an energy signal, or wave, and a receiving device, teams regularly collect data that are interpreted in terms of the depth, disposition/orientation, and composition of the ocean bottom and subbottom. Ever-increasing resolution of seismic data, coupled with equally precise geologic data to provide “ground-truth”, has revealed that (at least) the shallow subsurface beneath the seafloor is more dynamic, in all areas surveyed in detail, than was previously supposed.
The current work is restricted to a small portion of the upper continental slope of the northern Gulf of Mexico and to describing its heretofore undocumented geologic complexity. This portion of the Gulf has been a prolific producer of hydrocarbon fuels, so is of economic interest. The region figures prominently in the emerging story of gas hydrates and their possible role as an energy resource, in slope stability/instability, in greenhouse gas emissions, and as a resource for deep-sea chemosynthetic communities. Presented herein are the results of recent analyses of seismic and heat-flow data collected in Mississippi Canyon. Implications of these results to the larger issue of gas hydrates in passive continental-margin settings are addressed.
Background
Gas Hydrates
Gas hydrates are crystalline minerals composed of water and certain gases. They have been described extensively (Kvenvolden, 1993a, b; Max and Lowrie, 1992, 1996; Howell, 1993, Max, 2000) and are known to occur in the Arctic (Max and Lowrie, 1992) and in sediments underlying the world’s oceans (Fleischer et al., 2001, Kvenvolden 1993). The presence of hydrates can be inferred from seismic evidence such as bottom simulating reflectors (BSRs) or changes in seismic velocity (e.g., Hovland and Judd, 1988), or not (Lowrie et al., 1997). The petroliferous northern Gulf of Mexico is noted for its obvious absence of BSR’s, a characteristic it shares with other “active” passive margins with mobile salt and/or shale.
Hydrates form under well-defined ranges of temperature and pressure (Figure 1). For hydrate formation to take place, however, appropriate conditions of pressure and temperature must coincide with the availability of water and suitable gases. As with all buoyant fluids contained within sedimentary deposits, a particular form of hydrate exists only as long as prevailing temperatures and pressures remain within the ranges peculiar to that species. So hydrates, whose existence is so temperature- and pressure-dependent are believed to be ephemeral (Hovland and Judd, 1988, Rowe and Gettrust, 1993a,b), their previous presence sometimes being marked by features of explosive gas release such as pockmarks and mud volcanoes. This scenario fits with an evolving awareness that geology is far more dynamic than was generally believed only a few decades ago and that local and regional stress regimes can be extremely dynamic.
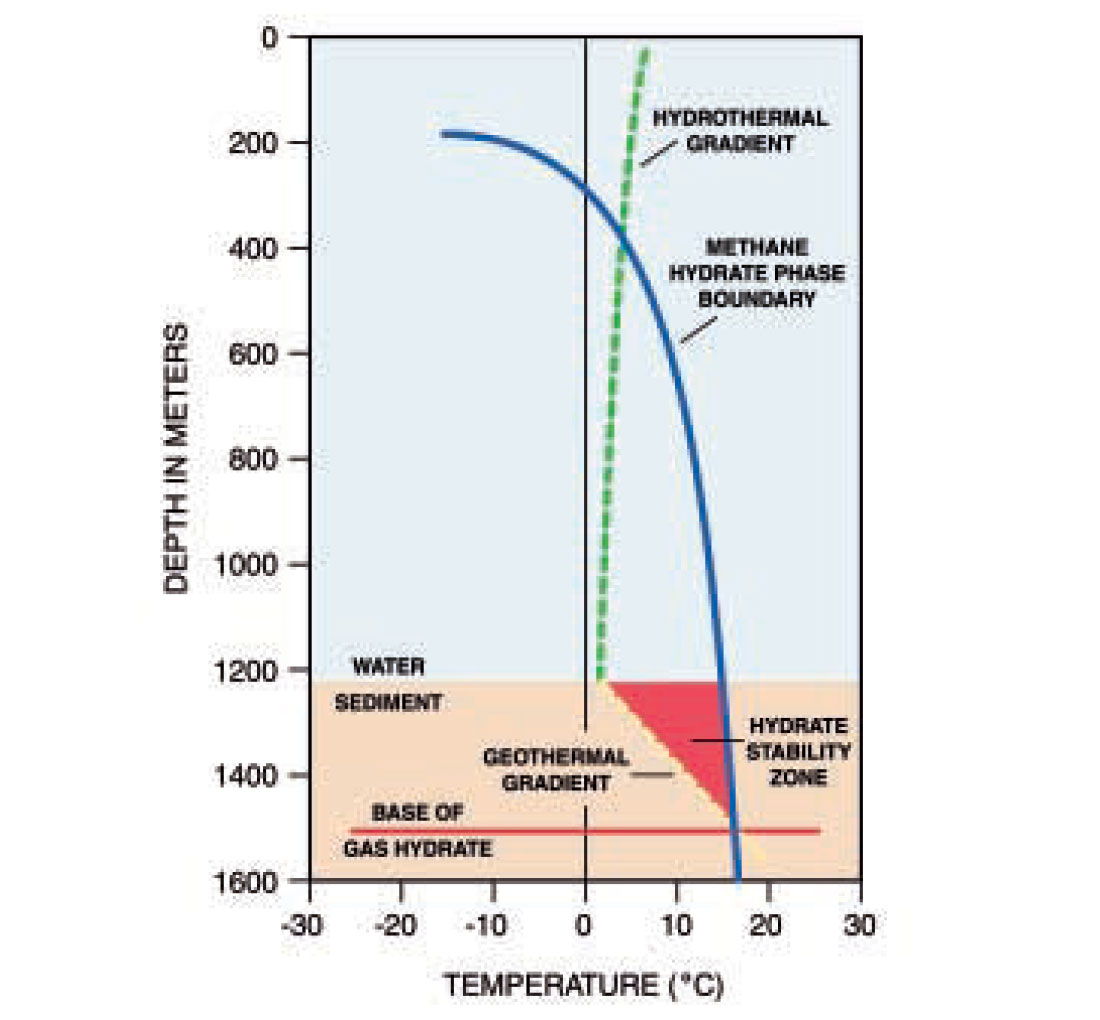
Within the World Ocean, seawater becomes cooler with depth, especially below the thermocline, and pressure increases by about an atmosphere for every ten meters of depth. Methane gas becomes hydrated at water depths of about 400m; methane with a 10% mixture of ethane will hydrate at depths as shallow as 100m (Baker, 1974). With increased depth, the thickness of the hydrate stability zone (HSZ) increases so that, in water depths of 3-4km, the HSZ can be up to a kilometer thick (see Figure 1).
Processes and their interactions along continental margins
Hydrates are known to exist in continental margin settings, yet to say with some assurance that there is a wide-ranging understanding of how hydrates impact a continental margin, as part of a geologic matrix, is premature. The following discussion is a proposed methodology with which to view and analyze a matrix of geologic processes, operating in linear and nonlinear ways, that could be operating in an environment such as the northern Gulf of Mexico. Since current knowledge (including detail) of the various processes and their interrelationships — both linear and nonlinear — is incomplete and evolving, using a matrix of processes, may elucidate reasonable inferences between and about these processes and improve an overall understanding of how continental margins operate.
The recent past, as measured by one and not more than two decades, has included much progress in discovering and understanding the structure of continental margins, both active and passive. Individual contributions are largely descriptive at this time (see Jackson et al., 1994 and Schultz-Ela and Jackson, 1996 for classical examples of descriptions and modeling) and there certainly is no clear formula of how geologic processes operate and interrelate over time. “Geologic process” is used here to refer to a single process, extracted from the totality of what occurs, geologically, in any given locale or region. For example, along the Louisiana upper slope, there are many processes going on simultaneously. A single geologic process, “deposition” (or “faulting” or “erosion,” etc.) can be extracted so that human minds, can concentrate on this single topic, “deposition”. In addition to facilitating human analysis, the conceptual purpose of segregating diverse processes is to facilitate analysis of each one. Research results from one process are then suitably organized so that study of their interrelationships may then proceed. This segregation of processes is an artifice done by humans for ease of study by humans.
Naturally there is the understanding that many other geologic processes are proceeding simultaneously within the region as the aforementioned deposition is occurring. For example, within the context of deposition, there may be variations in amount and type of deposition and even temporary cessations of deposition. It is important to note that it is not possible to predict how a given process will act over geologic time, i.e. how much will be deposited and in what manner deposition will occur during a sea level highstand (e.g. the Holocene) as contrasted with deposition during a sea level lowstand (e.g. a time of glacial advance and planetary cooling).
It is becoming increasingly obvious that geologic processes interact. The next concern to address is how the interaction proceeds. Does the interaction occur in a purely algebraic sense, with both positive and negative summations? In the case of deposition, the amount and manner of deposition appear to be important. If the amount deposited is minute and the depositing mechanism is by pelagic settling, there could not be any erosion of the substrate. If the amount is massive and the mechanism is via gravity-driven mass-wasting, a characteristic of dominantly, terrigenous deposition, there is a reasonable expectation that erosion can occur. These two independent variables, deposition amount and type, can be thought of as occurring in an additive manner, i.e. the more massive the amount deposited and the more energetic the mass-wasting, the greater will be the erosion prior to the subsequent deposition.
Suppose deposition is as described above, energetic and cumulative. The weight of accumulated deposits causes compression which leads to heat increase which, in turn, promotes hydrocarbon thermal maturation. If the temperature is lower than about 100°C, no hydrocarbons are generated but, if it rises above 100°C, maturation will occur. Therefore, a threshold will have been crossed and the evolutionary sequence can be called “synergistic;” once threshold conditions have been crossed, a totally new development comes into existence, one that could not have been predicted following a linear sequence. Thus interrelationships can be linear or nonlinear. The unexpected result in this case would be hydrocarbon generation. Other examples include mass-wasting events, catastrophic events that result in the rearranging of the geology and associated stresses that then commence to reaccumulate until the next threshold is crossed. In this case, thresholds are crossed and the “unexpected,” if a linear train of thought was being followed, occurs. In summary then, the interrelationships between processes may be thought of as occurring algebraically or synergistically.
A preliminary listing of geologic processes for the northern Gulf of Mexico should include the following: the formation of gas hydrates - possibly in several zones, one above another - and of associated free gas; organic material maintaining near-surface under-consolidation and potential hydrocarbon maturation occurring at multiple depth ranges, with the fluid hydrocarbons advancing in many possible directions and along different migration routes; sediment dewatering irregularly with depth; shallow water flows; smectite to illite conversion; dynamic geologic/tectonic components such as salt and/or shale diapirs or ridges; portions of the continental margin moving in various directions at varying rates; and the occasional creation of “shattered sediments” with a myriad of openings and closings of pathways through which fluids - both gases and liquids - migrate over minute to regional distances. All these are dynamic inputs into a final and complex synthesis. Each individual process should be studied independently. Sophisticated interrelating of all these processes can be developed as individual studies are completed and then considered together; first only two or a few processes evaluated, with the study becoming more complex and sophisticated as more processes are interwoven into an emerging geologic model.
Plate Tectonic Reconstructions
Plate tectonic reconstructions (Scotese, 1997; Smith and Briden, 1977; Smith et al., 1981, 1994) portray inferred paleoarrangements of continents at particular points in the long progression of geologic time. Evidence for these continental paleoarrangements is varied and draws from studies of stratigraphy, structural geology, paleomagnetism, paleontology, and geophysics, to name a few. Some forms are more compelling than others, some time periods are studied more intensely than others, and some data sets are more complete than others. Reconstructions usually show a researcher’s “best guess” at where landmasses above paleo-sealevel stood relative to each other, with the intervening sea floor lying between the continents. What is important in the present context are the possible paleosites for hydrate occurrence within the marine environment throughout the long history of our Earth (Figures 2a and 2b).
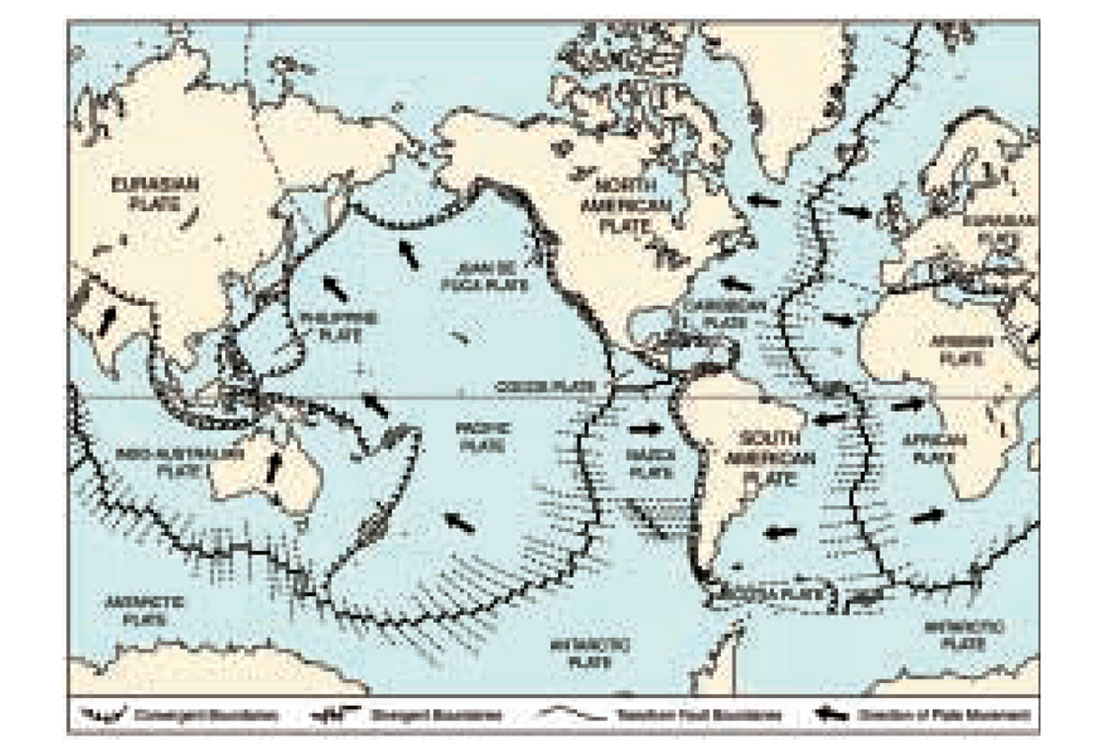
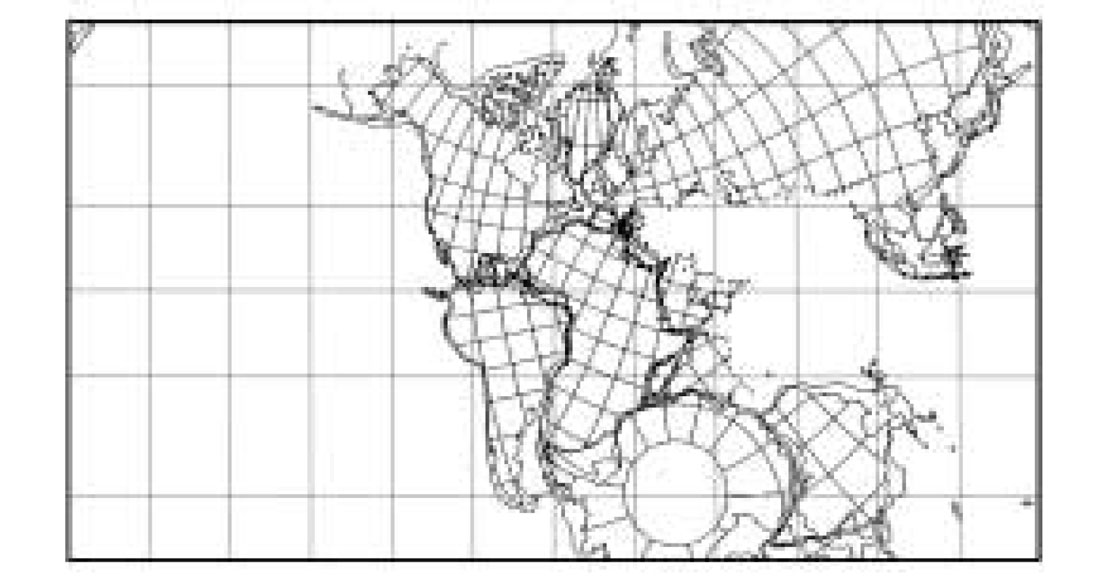
Environments conducive to hydrate formation have been created, destroyed, and modified throughout geologic time as new sea floor is created at mid-ocean ridges and destroyed along subduction zones (Figure 3), (e.g., Busby and Ingersoll, 1995). Environments are also modified by plate shearing (Isack et al., 1968), in concert with sediment deposition, throughout the marine environment. Thus, in a general sense, all hydrate occurrences and environments where they can accumulate are ephemeral, even if the time involved is measured in millions of years. Suppose conditions appropriate for accumulation occur within sediments along the flanks of a spreading ridge and also along continental margins where organic materials abound and where there are also conditions of thermal maturation suitable for hydrocarbon generation as well as for migration to accumulation sites/regions. Natural gases will migrate into the hydrate existence zone, will be converted into hydrates, and “freeze” into place. The hydrate volume will wax and wane as the amount of incoming and escaping gas varies with minor environmental fluctuations, and overall hydrate accumulation will persist.
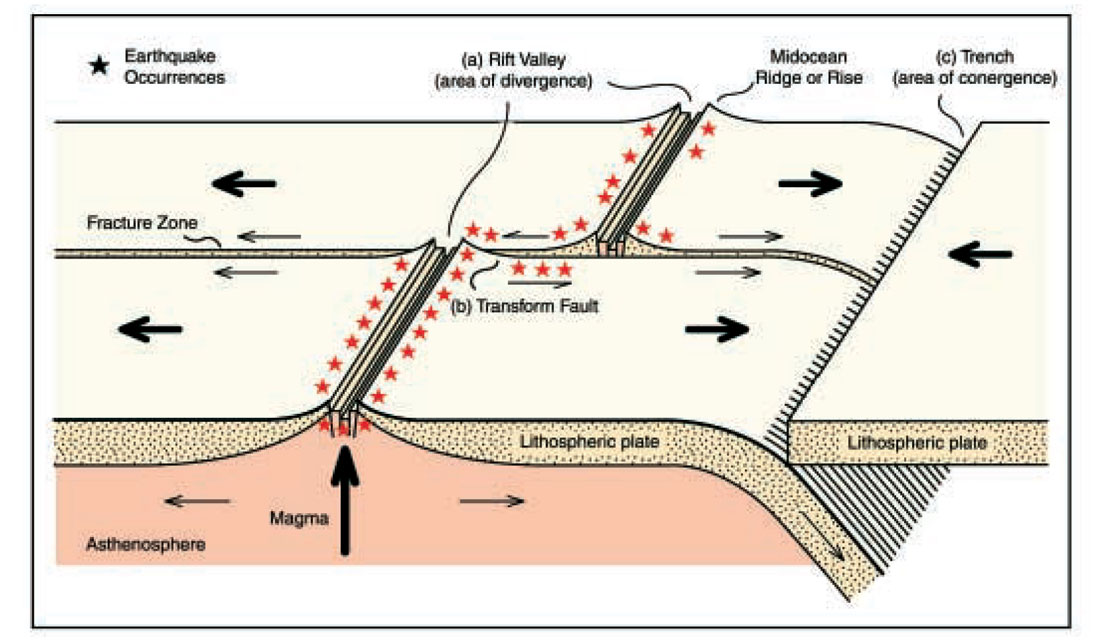
The “system” is composed of gas generation and migration, hydrate formation followed by hydrate decomposition and, lastly, gas escape into the ocean. Given the dynamic activity associated with contemporary hydrates, as observed in the northern Gulf of Mexico (Sassen et al., 2001; Roberts et al., 2001), it is not likely that individual molecules of methane and ethane, which have become hydrates, will remain at a specific site for a long time period, especially when residence time is measured in millions of years. The gas-to-hydrate-to-gas passage could occur in as short a time as a season within a year; present knowledge of transportation rates and the duration/periodicities of open migration routes are highly speculative.
Plate reconstructions by Smith and Briden (1977) and Smith et al., (1994) extend back to the Triassic. Scotese (1997) presents plate reconstructions that extend back to the Late Proterozoic (650 mya). These compilations suggest that classical plate tectonics have been operable for certainly a billion years. The question of how much further into the past were discrete and rigid plates moving on the planetary surface and interacting with each other is one that can not be answered from direct evidence; the ever-darkening fog that obscures the past makes interpretation increasingly tenuous as one moves farther and farther back in time. The very ancient rocks that have been studied repeatedly are interpreted to have originated at plate interaction sites such as rifting, shearing, and converging zones. These determinations do not themselves verify the existence of rigid plates nor do they oppose such an idea; the data are compatible with the notion of plate tectonics for as far back as has been determined, some 3.6 billion years. A definite answer may always elude searchers (Kevin C. Burke, University of Houston, Houston, Texas, telephone communication, 31 January 2002). For the purposes of the present discussion, the assumption is made that crustal processes were either plate tectonics or sufficiently like plate tectonics that the physics of temperature/pressure within the overlying oceans and on the surface of the plates would, given the presence of sufficient natural gases, allow the formation and eventual dissociation of gas hydrates.
These interpreted longevities of discrete emergent plates moving about the planetary surface assumes a sea floor covered by water. This sea floor has always been a catchment zone where sediments have been deposited, even with erosion over the emergent plates. Hydrocarbon generation appears to be a process integral to planetary geology (Gold, 1993) with hydrocarbons emerging from within the organic-filled sedimentary accumulations and from the rocky crusts. With there being a marine environment and sources of hydrocarbons, especially methane, it seems inevitable that hydrates should be an input to a geologic scheme based on plate tectonics. In the development of any complete description of plate tectonics and all the ancillary processes that can be occurring simultaneously in the sediments and the atmosphere above, the role of hydrates must be included.
High-Resolution Seismic Data
So-called “very-high-resolution” or “ultra-high-resolution” systems combine bandwidths of a few hundreds of Hertz to several kiloHertz with digital processing to resolve layer thicknesses on the order of decimeters. At this level of resolution, minor faulting has been interpreted at the sea floor and within the shallow subsurface (Rowe and Gettrust, 1993a, b). Using such systems, images of shallow faulting, hydrate accumulations, and pathways by which fluids reach the sea floor have been reported (McGee et al., 2001). Interpretations of such data locate and describe hydrates and the existence of paleo-hydrates at the sediment surface and shallow subsurface in unconsolidated sediments as evidenced by buried mounds and pockmarks. The age of these occurrences is often Pleistocene or younger (less than 2.6 mya, Morrison and Kukla, 1998), and almost certainly not older than Neogene (younger than a maximum of 26 mya).
Mississippi Canyon
The Mississippi Canyon is an offshore continuation of the Mississippi River channel, exposed during the great Pleistocene sea level lowstands. The canyon has been studied for a variety of reasons, included in various forms of marine research, and in data sets of several types. On the broadest scale, swath bathymetry and side-scan sonar data of the National Oceanographic and Atmospheric Administration (NOAA) have been employed to image the entire continental slope of offshore Louisiana (Figure 4). More locally, CMRET and the United States Geological Survey (USGS) of Menlo Park, California, have recorded seismic and acoustic profiles on both flanks of the Mississippi Canyon (along the tracks shown in Figure 5). In addition, heat-flow measurements have been collected on the canyon’s western flank on behalf of the CMRET.
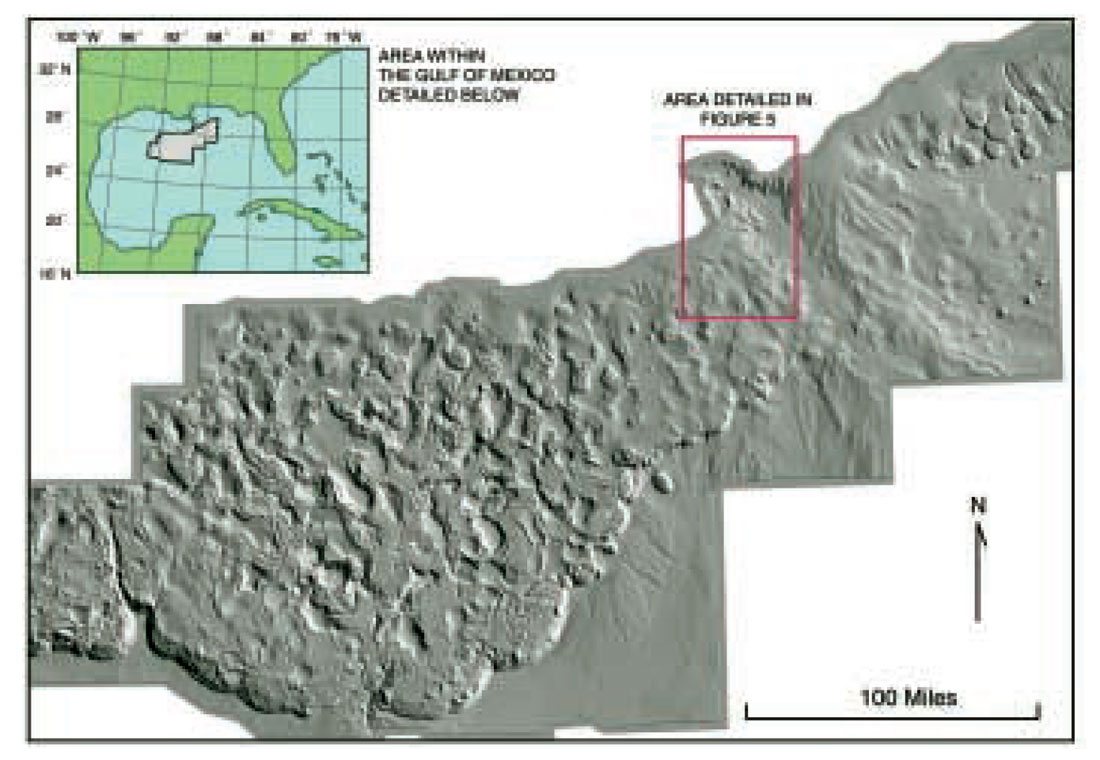
Mississippi Canyon Block 798 (MC798) in the northern Gulf of Mexico is situated along the upper continental slope of the Louisiana offshore and is an area of diverse geology and stress regimes. Over the past decade, MC798 Federal Lease Area has been of interest to the Center for Marine Resources and Environmental Technology (CMRET) of the University of Mississippi because gas hydrates, a research priority of the CMRET, have been sampled there (Neurauter and Bryant, 1989). There is no leaseholder from which to obtain permission for seafloor activities, and it is in reasonable proximity to ports in southern Louisiana and Mississippi. For these reasons, it is a convenient location in which to test systems and carry out hydrate research. It is from this base that the current research emanates.
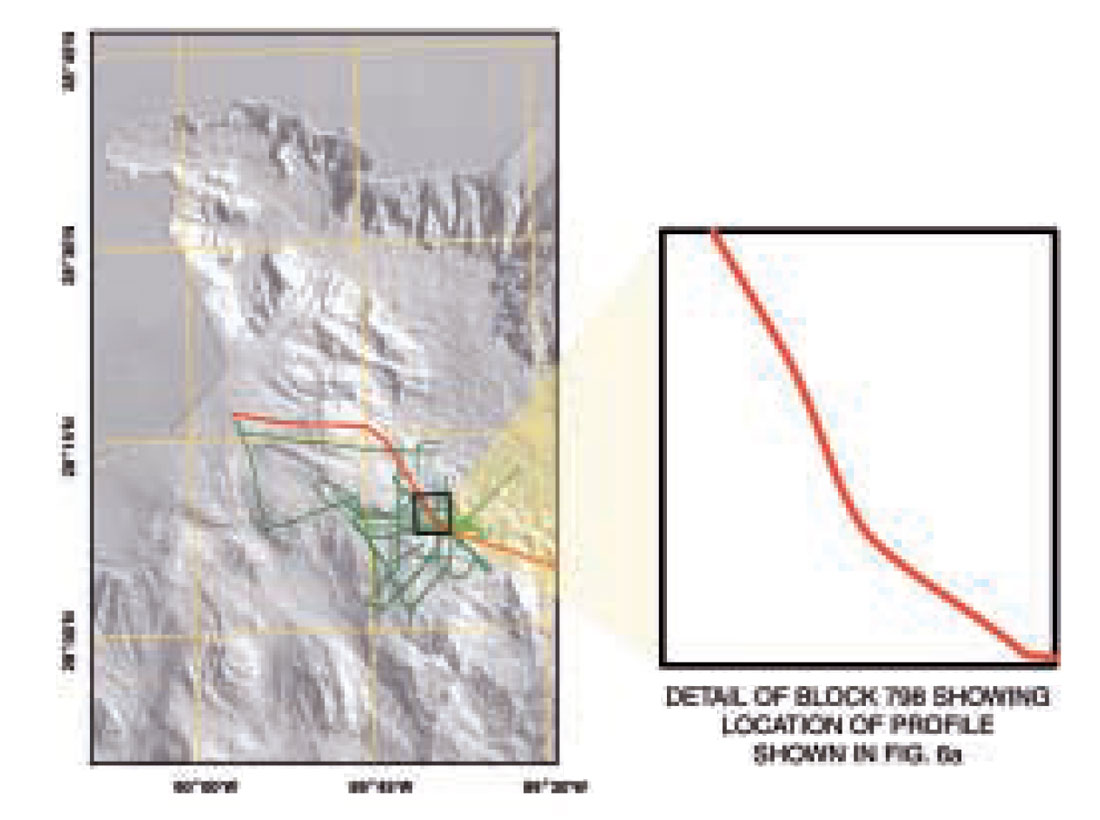
The remainder of this discussion addresses the geologic implications of data collected in 1998 from the western flank of the Mississippi Canyon in the northern Gulf of Mexico and what these results may imply in the broader context of gas hydrates along passive continental margins. The descriptions are part of a continuing study and results are preliminary. The data, the associated interpretations, and the understanding of the causative physics permit the casual inference that natural gas hydrates have a long history even though a sophisticated model for existent hydrates is not yet in place.
Methods
High-Resolution Seismic Data Collection Systems Used
For this investigation, three types of energy sources have been used to produce high-resolution, single-channel, reflection profiles from MC798 and its environs: G.I.gun (an airgun with air injection to suppress the bubble pulse), deep-tow boomer, and chirp sonar. The most conventional of these, the 35/35in3 G.I.gun, provides about 500m of penetration and resolves layers on the order of 3m. Very high-resolution deep-tow boomer and chirp-sonar data provide about 75m of penetration but resolve layers less than a meter in thickness and image features that are not seen in the G.I.gun data. The boomer provides information concerning reflection polarity but the chirp sonar does not.
Heat-flow data
The heat flow data were collected using a 3m violin-bow multipenetration instrument (Davis, 1988). Thirty-two stations were occupied along a NW-SE trending line. Water depths ranged from 850m to 1200m.
Digital Data Processing
The G.I.gun and boomer seismic data were processed digitally using methods described in McGee (2000). The principle steps were:
- Detrending: In order to avoid distorting the low-frequency portion of the seismic spectrum, the data were recorded without low-cut filtering. The result was that the raw data contained a significant amount of low-frequency offset. The offset was modeled on and subtracted from each trace using a method known as detrending that leaves the seismic spectrum relatively undisturbed.
- Source-signature phase conjugation: Water-bottom reflections from a number of traces were averaged to estimate a source signature for each profile. The phase spectra of these signatures were then used to remove the source phase characteristics from the traces. Both zero-phase and minimum-phase profiles were produced. The minimum-phase profiles were better for viewing seismic structure and the zero-phase profiles were better for picking arrival times and amplitudes.
- Spherical-divergence corrections: The data were corrected for amplitude losses due to spherical wave-front divergence.
Results
Mississippi Canyon Block 798
Three major physiographic provinces are represented in seismic data collected and analyzed in this study: the upper slope, an upper slope sub-basin, and a continued descent toward mid-slope depths. The sub-basin is delineated both up-dip and down-dip by salt structures, probably salt ridges of regional extent, that nearly reach the sea floor. Hydrates are known to occur in at least one seafloor feature in the block, the mud diapir cored by Neurauter and Bryant (1989).
Conventional high-resolution seismic data in and around MC798 show a wedge of relatively interpretable reflectors that widens progressively with depth. The widening wedge overlies a complex region several hundred milliseconds thick. Reflections within this region exhibit lateral variations of amplitude. Extrapolation of the heat-flow data held by the Mississippi Mineral Resources Institute, University of Mississippi, and collected by Trevor Lewis (consultant, personal communication, January 2001) suggest that the base of the hydrate stability zone (BHSZ) is located in the vicinity of the base of the complex region. Reflectors associated with the region’s base do not mimic the shape of the sea floor, so a true bottom-simulating reflector (BSR) does not seem to exist. Rather, reflectors in that vicinity are concave upward, their depth shallowing as they approach the salt, as would be expected of the BHSZ, due to relatively high heat conductivity within the salt. The variation in reflection amplitude may be due to irregular amounts of free gas being trapped against or within the BHSZ.
Seismic reflectors are hardly discernable and not at all interpretable beneath the complex region. This chaotic unit is interpreted to be attributable to absorption of the high-resolution seismic signal with depth.
High-Resolution Seismic Data in MC798
Of the three types of high-resolution zero-offset reflection data collected in and around MC798, the most conventional of these, 35/35in3 G.I.gun data (Figure 6a), provide about 500m of penetration. They exhibit what appear to be intrusions of salt and abrupt offsets of reflections interpreted as normal faulting. Some of the faults intersect the sea floor. It is not possible to discern from these data which, if any, of the faults are open to fluid flow. The overall concave-upward shape of the sea floor is suggestive of a canyon complete with a deeper flatfloored unit, possibly the axis of maximum flow, and an adjacent flood plain, all within the confines of two levee crests, the highest points along the profile. A wedge of relatively continuous reflectors is seen to thicken systematically with depth. This wedge overlies a region of complex seismic reflectors several hundred milliseconds thick. Reflections within this region exhibit lateral variations of amplitude.
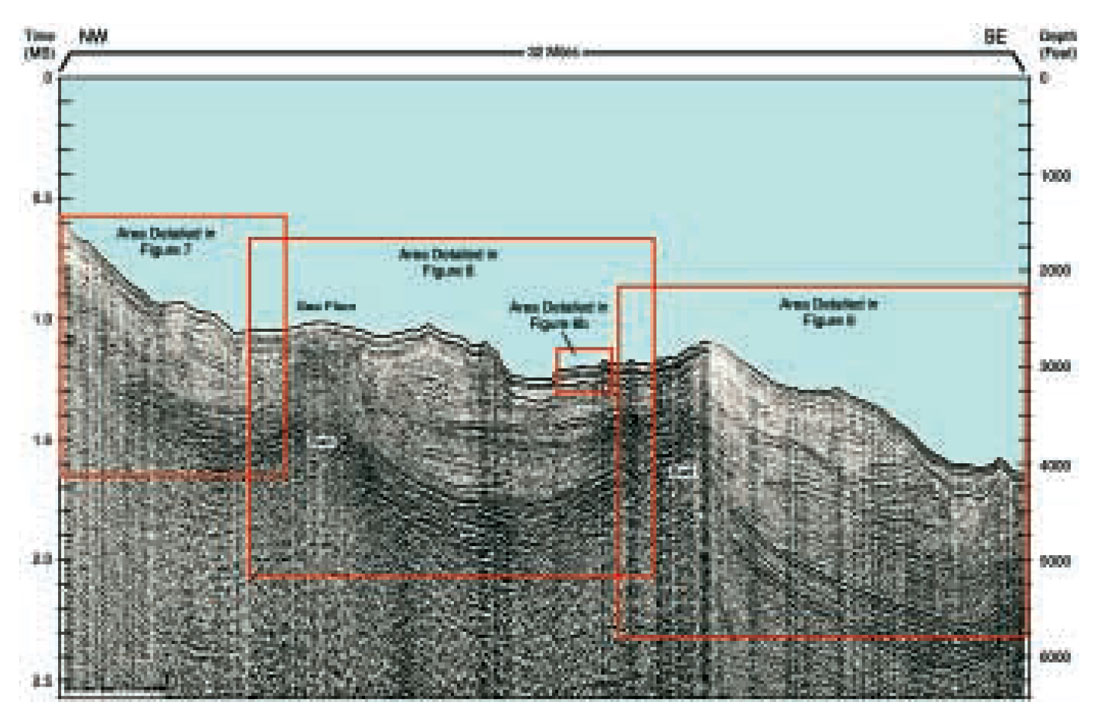
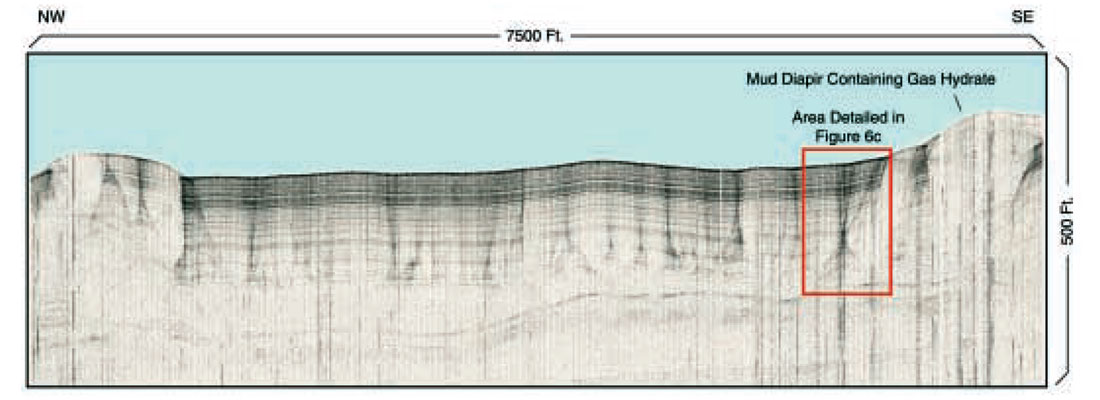
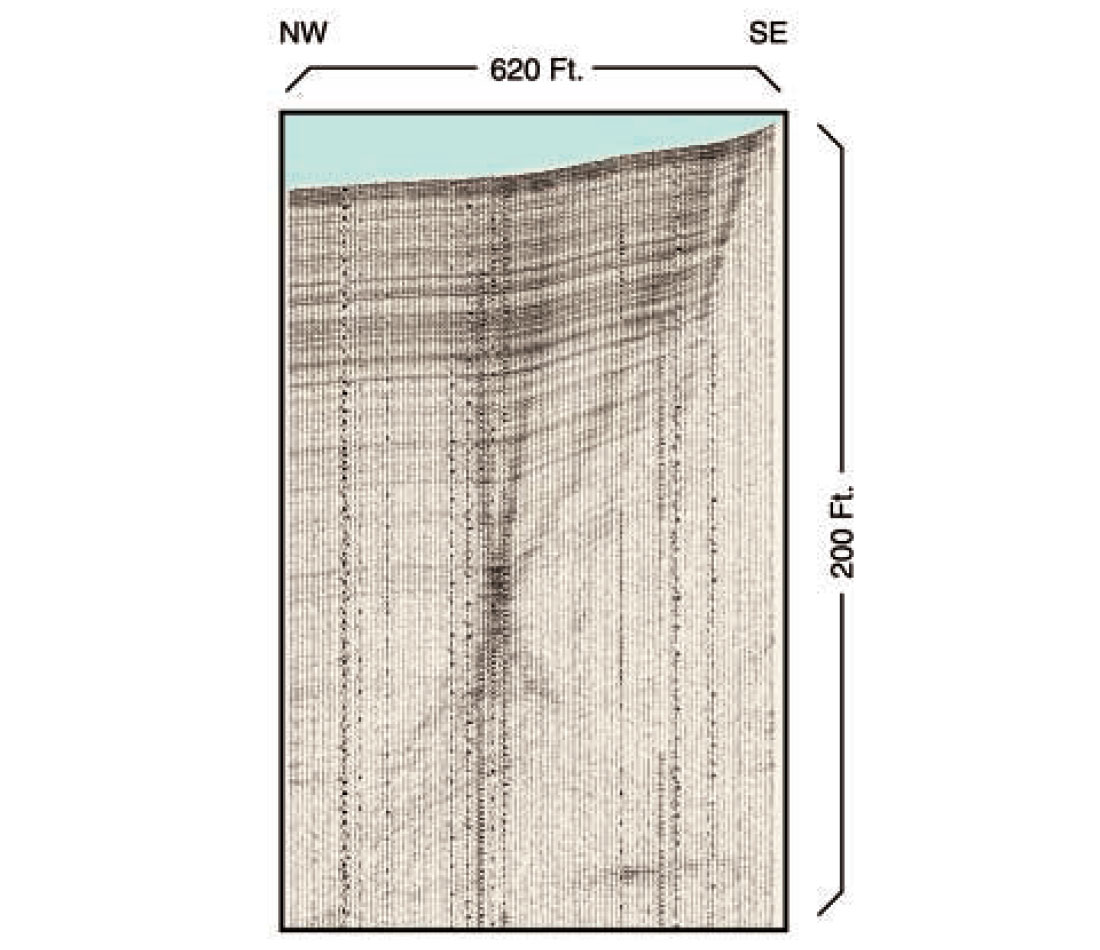
Very-high-resolution deep-tow boomer and chirp-sonar data provide less than 100m penetration but exhibit features that are not seen in the G.I.gun data. A section of 500Joule deep-tow boomer data (Figure 6b), collected at the same location as a portion of Figure 6a, shows vertically oriented structures of relatively higher reflection amplitudes interspersed with regions of generally lower reflection amplitudes. Also, the higher amplitude structures are rooted in the upper interface of a layer that appears to be devoid of internal reflections. The topographically high areas, generally devoid of internal reflectors, have the appearance of uplift yet do not seem underlain by salt, a common source of uplift along this continental margin. If these are the result of internal stresses within sediments alone, the reflectors lying immediately beneath the “uplifts” do not record similar up-bending (See Figures 6a and 6b).
This pattern of amplitude variation has been interpreted (McGee et al., 2001) as being due to the phenomenon of “reflection blanking” in the presence of gas hydrates. According to Dillon et al., (1994), this phenomenon can occur when hydrates are present on both sides of a reflector, causing an actual decrease in the impedance contrast, so that reflection amplitudes are lower than for those where hydrates are absent. With this interpretation in mind, the regions between the vertically oriented structures, as well as the layer devoid of internal reflections, appear to be relatively rich in hydrates while the structures themselves are relatively poor, perhaps being pathways where hydrates have dissociated under the influence of migrating fluids. An alternative explanation of the vertically oriented structures is that the regions of increased signal strength are interference patterns produced by interface geometry or inhomogeneities of physical properties, an explanation consistent with wave-front modelling (Warren Wood, personal communication) which may or may not be associated with the presence of hydrates.
The vertically oriented structure at the far right of Figure 6b is located in the vicinity of the anomalously high heat-flow measurement discussed previously. An enlarged view of it (Figure 6c) shows the intricate amplitude variations that comprise these structures. The areas of stronger reflector amplitudes, suggested as hydrate-poor and possibly zones of some fluid-flow, also mark a change in inclination of adjacent reflectors. A reflector change in dip could mark different stress fields, possibly even denoting a flexure (not a fault with actual movement, yet a flexure with bending).
Seismic Descriptions Along a Dip Traverse
A seismic (35/35in3 G.I.gun) traverse across MC798 is described below in segments keyed to the block’s three physiographic provinces (upper slope, slope sub-basin and middle slope). The descriptions are presented as part of an on-going study and should be regarded as preliminary.
A traverse of the upper slope is shown in Figure 7 where a reflection, possibly marking the BHSZ, is seen clearly across the deeper two-thirds of the slope. That reflection breaks abruptly and becomes much more diffuse in the up-slope direction. Also, the seismic character above and below the break shows distinct changes. The disorganization of reflection character up-dip from the break appears to characterize energetic mass-wasting. Directly above the possible BHSZ lies an orderly series of reflectors, suggestive of much less energetic deposition and representative of a definite periodicity to the deposition. Farther above, the reflectors begin to exhibit some irregularity, another indication of a change in the depositional regime. Along the sea floor and in the shallowest sub-surface minor irregularities are seen. These are caused by minute normal faults and indicate active down-slope extensional tectonism. The seafloor slope changes abruptly in the middle of the illustration with an interpreted debris pile at the intersection of the two seafloor trends. Such geologically rapid changes in debris cover alter the underlying stress and temperature conditions. Confusion of reflectors deriving from faulting and mass-movement of sediments distort stratal surface and thus preclude interpretation of any continuous surface through the section. Therefore, application of a traditional sequence stratigraphic interpretation to this section is not an option (Vail, 1987, Haq et al., 1987); the greater detail in these high-frequency data may cause the difficulty.
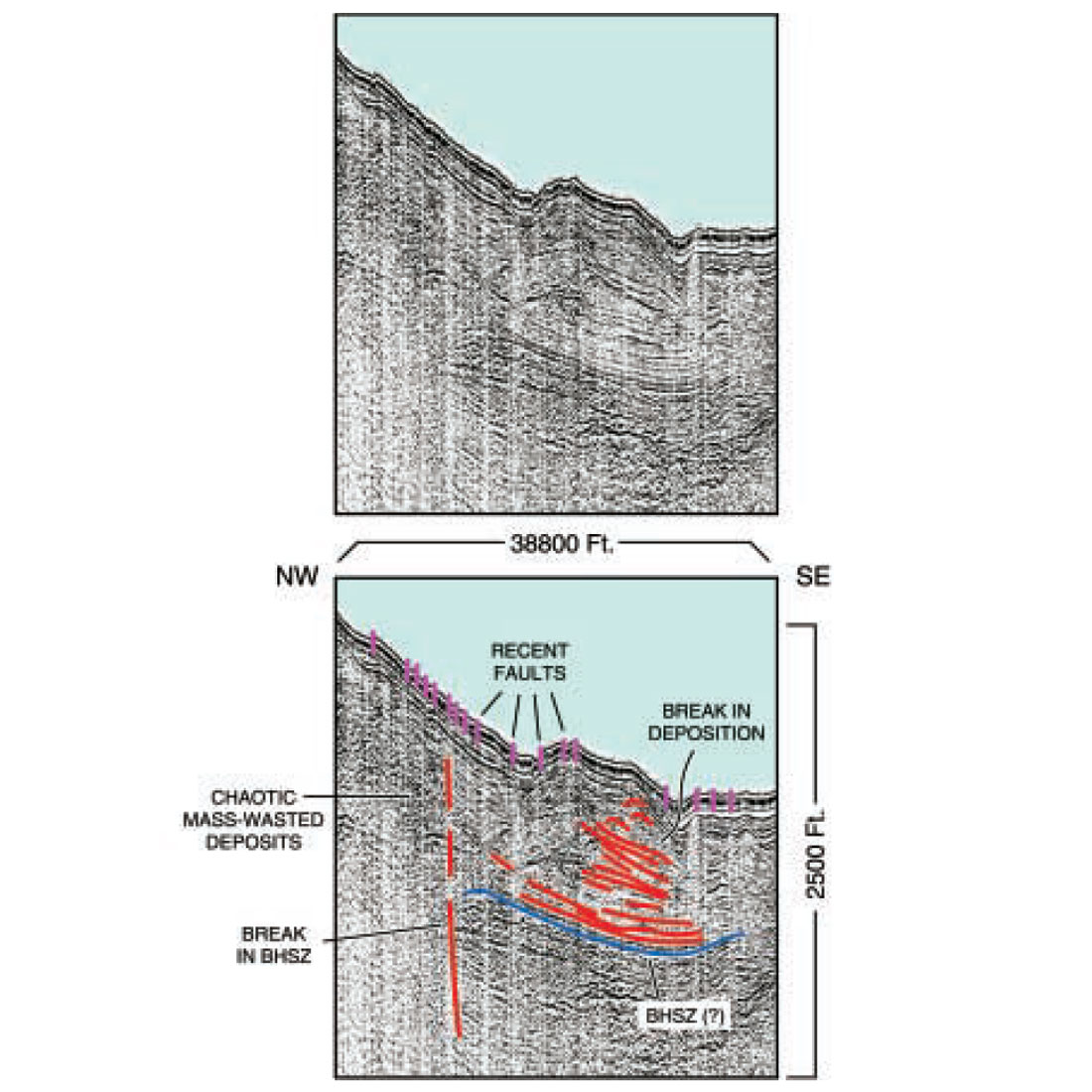
The next described section, Figure 8, spans the upper slope sub-basin. This sub-basin is delineated both up-dip and down-dip by salt structures that appear to reach nearly to the seafloor. The tops of these salt structures are irregular and apparent interfingering between salt and sediments further obscures the contact. The seafloor profile is irregular yet does not show any trend in slope. Apparently, the bordering salt structures (probably salt ridges of regional lateral east-to-west extent) are uplifting this portion of the upper slope locally, while the resultant basins that form between the salt structures serve, simultaneously, as areas of sediment catchment. This sub-basin was the site of the heat-flow measurements that were extracted, illustrating that the BHSZ would be about 500m below the sea floor. A reflection visible across the central portion of Fig.8 is near the expected depth of the BHSZ and appears to be negative in polarity, also as expected. The BHSZ(?) reflection continues across the basin but weakens and shallows as it impinges onto both salt structures. The BHSZ(?) shows appreciable variations in reflection strength; a casual surmise being that there are major variations in density/amount of actual hydrates and in accompanying free gas trapped beneath the hydrate. Should there indeed be such variations, they would produce definite differences in buoyant forces across this expanse of the sub-basin, contributing to continental margin weakness.
In Figure 8, the BHSZ(?) reflection appears to traverse through the salt itself and continue up-dip; such apparent continuity may be fortuitous only given the abundance of reflectors. It is not likely that hydrates exist within the salt because their formation would be inhibited, so the BHSZ(?) reflection and the salt are probably at different locations in three dimensions, at least one of the locations being out of the plane of the section. Within the sediment mass, there are breaks of continuous and discontinuous reflectors adjacent to each other. The continuous reflectors mark uniform deposition and the discontinuous reflectors are from high energy, erosive mass-wasting that presumably removed the pre-existing continuous deposition.
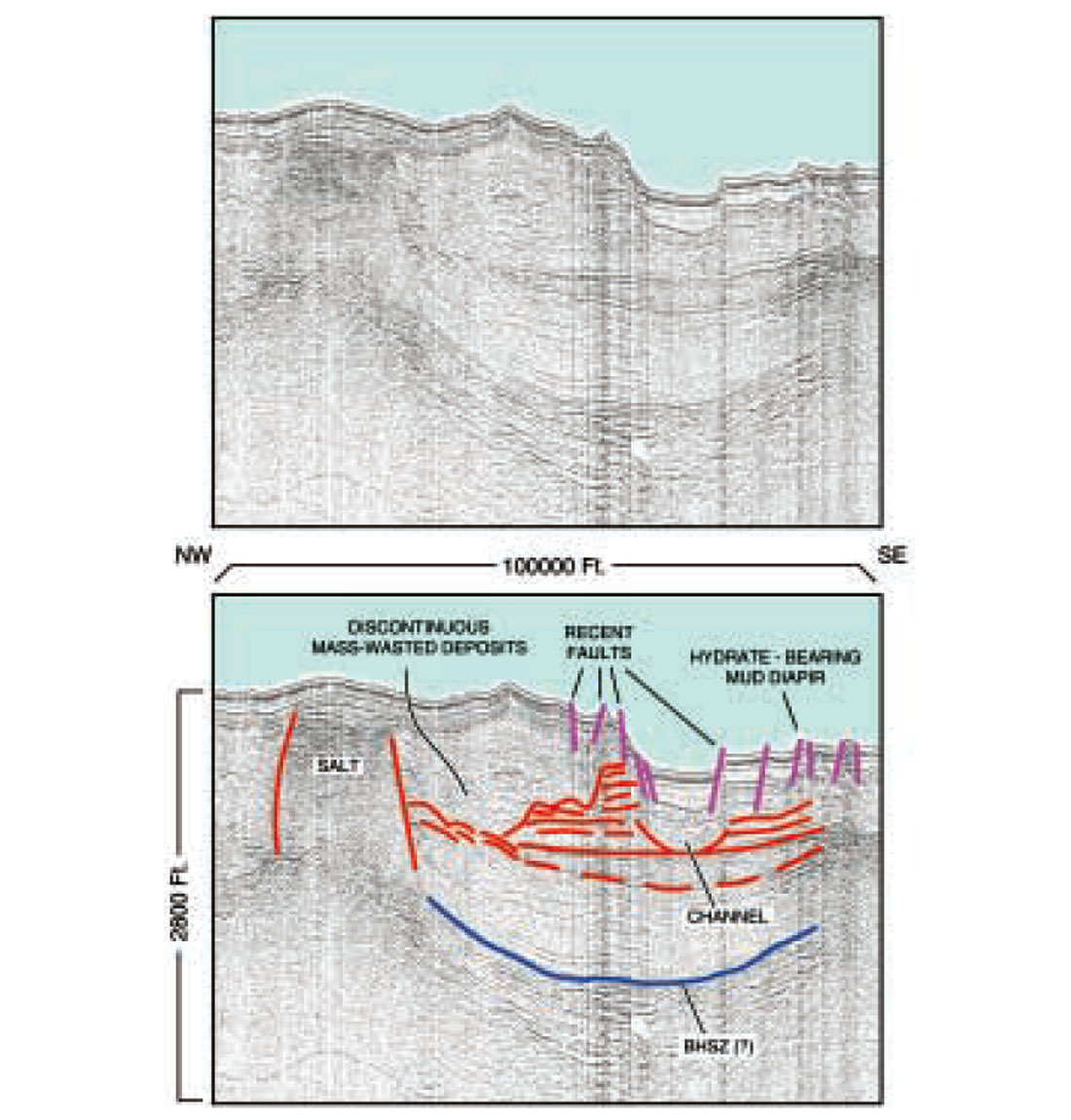
Further downslope, an irregular sea floor is seen in Figure 8. The sea floor is marked by abrupt bathymetric breaks that are easily interpreted as the result of relatively recent normal faulting. Farther into the subsurface there are several concave upward reflectors of varying sizes; these reflectors appear to mark the outline of submarine canyons. Approximately midpoint through the seismic section is a zone of complex and disorganized reflectors that appear as the consequence of a weak BHSZ. This sea floor was the site of the collection of a series of cores and heat flow measurements.
One of the indicated seafloor faults is the location of a ten-fold anomalous heat flow measurement. Other cores were taken near and adjacent to some of the other bathymetrically marked faults. These latter cores contained crudely uniform heat flow values, varying locally with doublings and lesser amounts of change. The fault marked by the ten-fold heat increase was apparently open and permitted the migration of hot fluids from depth, those fluids venting into the water column. Such heats could represent fluids whose warming may have occurred at depths of a kilometer or two (regional thermal gradients cluster about a value of 2.3- 2.5°C/100m; uncomplicated thermal gradients could yield a simplified casual depth of origin calculation). The bandwidth of these data does not provide the detail required to show that the fault is open to fluid migration. Adjacent to the potentially open fault is the hydrate-bearing diapir reported by Neurauter and Bryant (1989). That the hydrate had not been decomposed by the nearby open fault suggests a complex thermal distribution. In fact, there is evidence that a stream of dense brine about a meter thick is flowing across the seafloor (Trevor Lewis, Consultant, reporting on heat-flow analysis, January, 2001).
In Figure 9, the basinward salt structure is represented and the upper slope commences to descend again toward mid-slope depths. In this segment, the strength of the BHSZ(?) reflection is less. Whether there could be less hydrate and/or free gas in this area can not be verified. Within the sediment-dominated section there is evidence of block rotations. Regional structural weakness may once again be apparent as the slope descent commences anew. Once again, there are small faults that intersect the seafloor, indications of active tectonism.
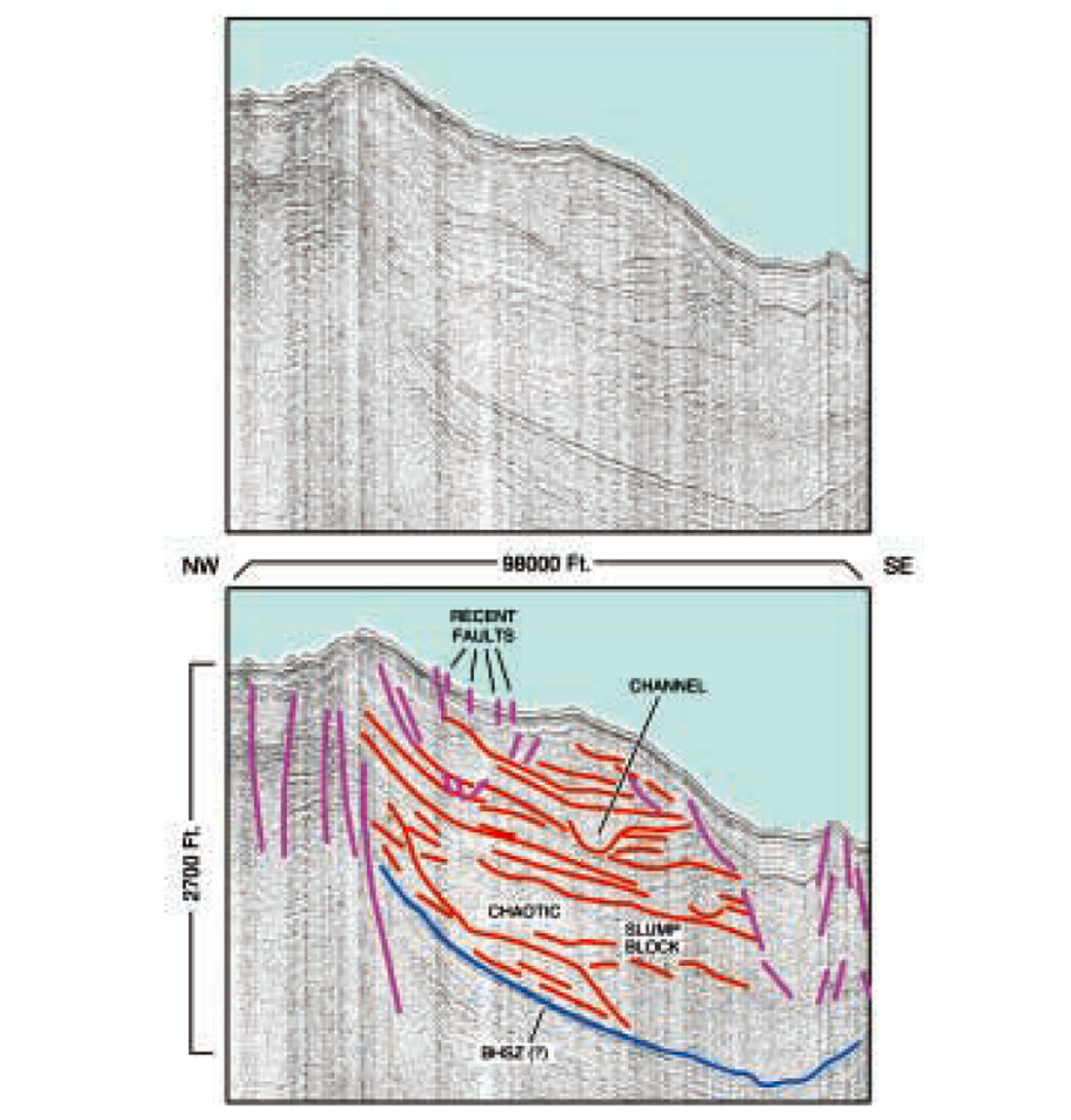
Farther downslope, the BHSZ(?) continues to be relatively weak as compared with the slope sub-basin and upper slope. The juxtaposition of continuous and discontinuous reflectors indicates two different depositional patterns. The continuous reflectors indicate deposition that was probably ubiquitous, although intermittent. The discontinuous reflectors indicate that high-energy mass-wasting would have comprised events that were locally catastrophic. These events would have eroded material previously in place and deposited younger material in the eroded zone. The existence of a local diapir-like structure within the entirely sedimentary unit argues that, while the overall slope is a province of extension, there appear to be localities where the generally extensive, gravity-driven, down-slope motion can be converted into minute compressive environments.
Conclusions
Two disparate ideas are expressed here: that hydrates have been forming and dissociating for a long time, possibly for billions of years, within the context of plate tectonics, and that contemporary hydrates along the northern Gulf of Mexico continental margin, represent a complex, dynamic and geologically young environment. These two proposals draw from widely different time periods for viewing geologic phenomena. The purpose of the speculation of hydrate longevity is to invite the incorporation of hydrates into future geologic summaries and to request an active search for evidences of paleo-hydrate accumulations and their dissociation, a suggestion analogous in spirit to the request two decades ago to seek out vestiges of paleo-earthquake activity. The details of hydrate existence over such immense time ranges are not known, yet it seems reasonable to suggest that they have indeed existed.
Preliminary descriptions of seismic sections that traverse the upper slope of the northern Gulf of Mexico, namely the area of MC798 and adjacent lease blocks, have been described with special emphasis on features that denote structural weakness and the impact of dynamic processes. It is supposed that gas hydrates have been intimately related to those features.
The descriptions, while applying directly to sites in and about MC798, are actually quite general and could be applied to numerous, if not a majority of, geologically similar environments across the central and adjacent portions of the western northern Gulf of Mexico continental margin. By extension, application of these findings and speculations to similar margins elsewhere in the world - SE Brazil, Angola, Nigeria, Mediterranean Egypt – may aid in furthering both historical and contemporary understanding of those environments.
A “final” and generalized opinion that derives from analyses of these sections from the Mississippi Canyon is that the same processes described here likely weaken many sections of all these various margins. Such an observation does indeed lead to the conclusion that these types of continental margins are surprisingly complex, dynamic, and structurally weak with both linear and nonlinear interactions occurring. The fundamental weakness of a regional description such as this is that it is based entirely on remotely sensed high-frequency data in the absence of “ground truth” from core samples or, better yet, from monitoring stations that record continuously the dynamic environment represented here.
Acknowledgements
The seismic data presented herein were recorded by the U.S. Geological Survey (Menlo Park) and processed by the Center for Marine Resources and Environmental Technology (CMRET) of the University of Mississippi. The illustrations for this manuscript were ably prepared for publication by Paul Mitchell; thanks are extended.
Join the Conversation
Interested in starting, or contributing to a conversation about an article or issue of the RECORDER? Join our CSEG LinkedIn Group.
Share This Article