1. Introduction
For many people the name Tibet is associated with a mysterious and distant land inhabited by monks, nomads and yaks. Made popular by recent films, and with a unique culture, this perception of Tibet is certainly true. However, Tibet is also a natural laboratory where scientists can study what happens when continents collide and build the world’s highest mountains. These collisions were responsible for the assembly of the modern continents, beginning more than four billion years ago. Thus studies of ongoing continent-continent collisions give a vital insight into the history of older continents such as North America.
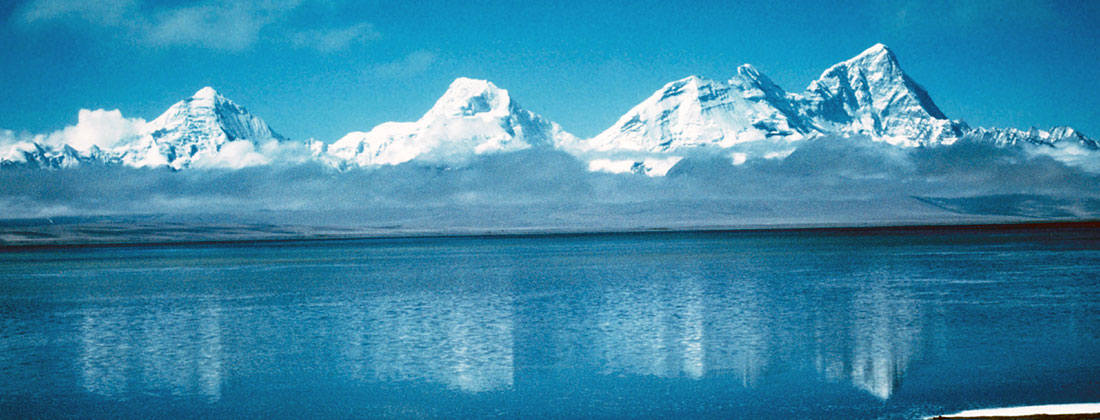
It is universally accepted that the Tibetan Plateau has been formed by the collision of the Indian and Asian plates. Paleomagnetic data allows India’s northward journey to be reconstructed, and the best estimate is that the Tethys ocean closed from west to east beginning 53Ma ago (Leech et al., 2005). Continental crust is too buoyant to be subducted so the collision has thickened the crust to form the largest topographic feature on the surface of the Earth. The Indian Plate continues to move northward at 4 cm per year and has built a plateau with an average elevation of 5 km over an area 2000 km east-west and 1000 km north-south (Figure 2). However, the mechanisms that formed the Tibetan Plateau are still not fully understood. In this article, previous geological and geophysical studies are summarized. The results of modern geophysical studies are summarized and their tectonic implications discussed.
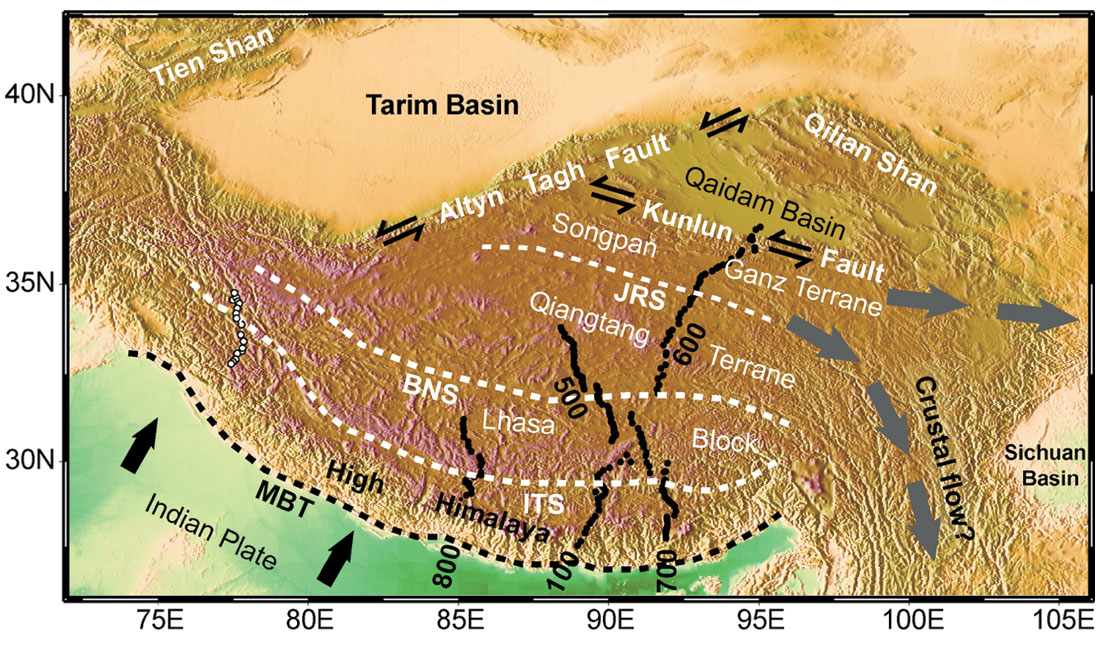
2. Early investigations in Tibet
The first geophysical study of the Himalaya was the geodetic work of George Airy. During a survey of India, precise observations of the position of the stars were needed and a plumb line was used to accurately define the vertical direction. Corrections were made for the anticipated gravitational attraction of the Himalaya, but careful data analysis showed that this attraction was less than expected. Airy interpreted this observation with the hypothesis that the Himalaya were underlain by a low density crustal root (Airy, 1855; Figure 3). He proposed the concept of isostasy and his calculations predict a crustal thickness in southern Tibet of 80 km. This thickness is twice the global average and has been confirmed by modern seismic reflection surveys during the INDEPTH survey. However, the cost of the seismic survey was orders of magnitude greater than the early geodetic work, and the cost efficiency of modern geophysics could be debated.
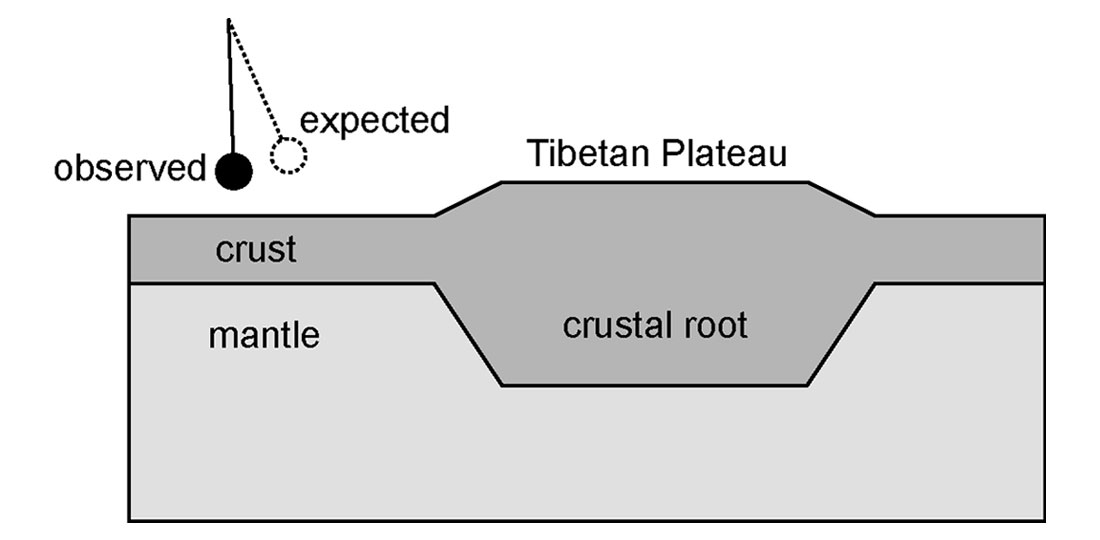
3. Geology of the Tibetan Plateau and geodynamic models
There are many ways to generate a crust that is twice the normal thickness. An early suggestion was that of Argand (1924) who proposed that India had completely underthrust the entire 1000 km north-south extent of the Tibetan Plateau. With the recognition of the link between ancient mountain belts and plate tectonics, Dewey and Burke (1973) suggested a very different model with the double thickness crust being formed by distributed north-south compression. Computer modeling of this type of deformation accounts for a number of observed features (England and Houseman, 1986).
Geological mapping provides information that is vital for differentiating between the models such as those listed above. Major progress has been made in mapping the geology of the Tibetan Plateau over the last three decades (Yin and Harrison, 2000). The Tibetan crust is composed of a series of terranes that were accreted to the southern margin of Asia over the last 500 million years. From north to south these are the Songpan-Ganze Terrane, Qiangtang Terrane and the Lhasa Block and their boundaries are delineated by ophiolite belts. The south side of the Lhasa Block is delineated by the Indus-Tsangposuture (ITS), which defines the location where the Indian and Asia continents collided and closed the Tethys Ocean. The Gangdese batholith dominates the southern Lhasa Block and was the result of magmatism generated by the subduction of the Indian Plate prior to collision (Yin and Harrison, 2000). Further south a re the high peaks of the Himalaya, generally composed of overthrust sedimentary rocks, but also with major sequences of granites that originated in crustal melting (Le Fort et al., 1987). Other tectonic processes may have contributed to the evolution of the Tibetan Plateau and Himalaya. While there is no active volcanism in Tibet today, mafic volcanic rocks younger than 13 Ma are found in Northern Tibet. These rocks may have originated when part of a thickened lithosphere broke away and initiated localized convection of the asthenosphere (Molnar et al., 1993).
Neotectonic studies have shown that thrust faults continue to be active today at low elevations in the Himalaya. However, the tectonics at higher elevations are characterized by normal faulting that leads to crustal extension in a series of north-south trending rifts (Armijo et al., 1986). The uniform elevation of the Tibetan Plateau represents a dynamic balance between ongoing uplift and erosion / tectonic collapse. Locally the topography can be higher (8 km in the Himalaya) but over most of Tibet the surface is flat and 5 km above sea level. Ongoing collision cannot raise the Tibetan plateau higher, but the horizontal extent increases. In northeast Tibet, the plateau still rises in the active fold-and-thrust belts of the Qilian Shan and Daxue Shan (Meyer et al., 1998; Bedrosian et al., 2001).
Geological mapping in south-east Asia has also revealed that the India-Asia collision has caused significant horizontal motions on major strike-slip faults, such as the Red River fault. Tapponier et al., (1982) used plasticine modeling to show that this extrusion is the consequence of a free boundary on the eastern side of the collision zone. This extrusion continues today as confirmed by detailed global-positioning system (GPS) data (Zhang et al., 2004) and may have absorbed as much as 30% of the convergence (Replumaz and Tapponnier, 2003). In Northern Tibet, extrusion occurs on the Altyn Tagh Fault and Kunlun Fault (Bendick et al., 2000; Lin et al., 2002). It has also been suggested that subduction of Asian lithosphere may take place southwards beneath Northern Tibet (Willett and Beaumont, 1994). Geological studies have given many insights into the timing and dynamics of the collision. At least 1400 km of north south convergence has occurred and mass balance calculations have been used to evaluate the roles of the various processes that have led to the rise and fall of the Tibetan Plateau (Yin and Harrison, 2000). However, a complete understanding of the tectonic processes requires geophysical imaging of the crust and upper mantle.
4. Early geophysical studies
Access to the Tibetan Plateau has always been logistically difficult. Airy overcame this problem by making his observations without even entering Tibet. Prior to the 1950’s Tibet was de facto independent and access to foreigners was very limited. The Chinese annexation of Tibet in 1950 prevented serious scientific investigations for nearly 30 years. Studies in the Indian Himalaya revealed an elevated heat flow (Shanker et al., 1976) that was interpreted as localized partial melting by Gupta et al., (1983). In the 1980’s Chinese scientists began systematic geological mapping and geophysical studies were begun. Co-operation with French scientists in the 1980’s led to the collection of seismic data in Tibet that showed that the crustal thickness in Southern Tibet was in excess of 70 km (Hirn et al., 1984). Magnetotelluric data showed that the crust was characterized by zones of low electrical resistivity (Pham et al., 1986). Combined with the elevated heat flow it was suggested that partial melt was present in the Tibetan crust (Franchetau et al., 1984). Are cent summary of all geophysical results from the Himalaya and southern Tibet is presented by Klemperer (2006).
5. INDEPTH seismic reflection data
A more detailed phase of geophysical exploration began in the early 1990’s. The Consortium for Continental Reflection Profiling (COCORP) had been successful applying industry seismic techniques to tectonic studies in the United States. In the 1990’s this group began to apply these techniques to studies outside the United States. The INDEPTH project (International Deep Profiling of Tibet and the Himalaya) used Chinese seismic crews to collect 16-fold common mid-point data using dynamite sources on a profile crossing the High Himalaya (Figure 4). Despite problems with drilling shot holes, and wind noise, high quality data were collected up to 25 s two-way travel time. This survey showed a prominent north dipping reflection that could be projected southwards to the location of active underthrusting in Nepal. This feature was termed the Main Himalayan thrust (MHT) and was interpreted as the top of the underthrusting Indian Plate (Zhao et al., 1993). Based on the success of this project, additional funding was received for an extension of the profile to the north across the Indus-Tsangpo suture (ITS). Rugged terrain did not permit continuous profiling and there w e re gaps in the data coverage. Wide-angle recording of refracted arrivals was utilized to fill some of these gaps, with shots up to 1000 kg that were recorded to offsets of 200 km. The new CMP reflection data showed that the MHT could be traced northwards, but not beyond the ITS. Beyond this point, the seismic structure changed and a series of bright spots was observed (B1 and B2 in Figure 6). The high amplitude and negative polarity of these bright spot reflections strongly suggest a fluid phase (Brown et al., 1996). Partial melt or aqueous fluids are the most likely fluids and amplitude-versus-offset (AVO) analysis has been used in an attempt to discriminate between the two (Makovsky and Klemperer, 1999). Their results suggest that the most likely explanation is a thin layer of aqueous fluids overlying a thicker zone of partial melt.
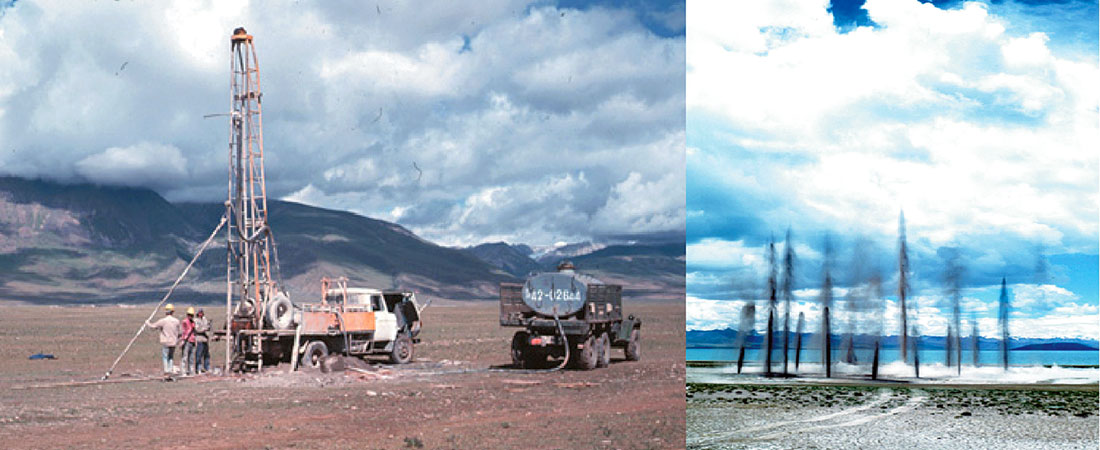
6. Magnetotelluric exploration in Southern Tibet
The possibility of fluids in the Tibetan crust led to the inclusion of other geophysical techniques in the INDEPTH project. This initially included passive seismic techniques using earthquakes as energy sources (Kind et al., 1996) and magnetotelluric (MT) exploration was included beginning in 1995. Previous Chinese and French MT data collected in southern Tibet had suggested that low resistivity zones were present in the c rust. However, these surveys did not use modern instrumentation or processing, and did not collect sufficient data to give reliable 2-D resistivity images. The western collaborators in the 1995 fieldwork were the University of Washington (UW) and the Geological Survey of Canada (GSC). In cooperation with the China University of Geosciences in Beijing (CUGB), the INDEPTH project collected MT data on a profile (100-line, Figure 2) that was coincident with the INDEPTH seismic reflection data at 90°E. The magnetotelluric (MT) data are recordings of the time variation of the Earth’s magnetic and electric fields, and MT data processing converts these data into estimates of apparent resistivity in the frequency domain (Simpson and Bahr, 2005). Since lower frequencies penetrate deeper into the Earth, a resistivity-depth profile can be derived from recording data at a range of frequencies. To record the necessary frequencies, fieldwork generally requires two types of MT instrumentation. The CUGB group used a broadband Phoenix Geophysics V5 system to collect data for crustal imaging (500 – 0.001 Hz) with induction coils for the magnetic field measurements. The UW-GSC group measured low frequency data (1 – 0.0001 Hz) with a system based on a flux gate magnetometer that extended coverage to lower crustal and upper mantle depths.
This survey was my first visit to Tibet and I spent 2 months working there in 1995. In subsequent years I have spent 8 months in Tibet during 4 field seasons. Geophysical research in Tibet is challenging in many ways. The high altitude makes fieldwork very strenuous, and the lack of roads makes travel very time consuming. In addition, cultural issues require endless patience in negotiating contracts and running fieldwork on a daily basis. Living conditions in Tibet are generally primitive and scientists who expect running water and other plumbing do not often return. Long-period MT systems collect data in the frequency band 1–0.0001 Hz and are operated for a month at each recording location. A lot of effort was put into instrument security and the usual strategy is to hire guards from the nearest village and pay them to camp close to the instrument. This process was made easier once I had learnt some very basic Tibetan, and developing friendships with Tibetan villagers was a very rewarding aspect of the work. At one location the guards were over-zealous and hung their iron cooking pot a few metres from the magnetic sensor. This resulted in some interesting magnetic signals being recorded.
Magnetotelluric data on the 100-line profile were converted from the frequency domain to true depth using an automated 2-D inversion process. This process is non-unique, and a common strategy is to require that an acceptable resistivity model fits the measured MT data and is also spatially smooth. Thus the resistivity model in Figure 6 is the simplest model that fits the field MT data. In this model it can be seen that the underthrusting Indian plate has a high resistivity, as expected for old, cold lithosphere. North of the seismic bright spot B1 a prominent zone of low resistivity is imaged with the top at 20 km depth. The top is coincident with the seismic bright spots, supporting the fluid explanation for both geophysical anomalies. A quantitative analysis requires 5-7% partial melt to account for the observed electrical resistivity. Seismic attenuation in this layer explains why the MHT reflection is not imaged further north. This melting is initiated by radiogenic heat production in a thickened crust. Unsworth et al., (2005), showed that these amounts of partial melt are sufficient to lower the viscosity of the crust by an order of magnitude, which is sufficient to permit crustal flow in a narrow channel as proposed by Beaumont et al., (2001). This flow is driven by rapid erosion and transports rocks from the midcrust to exposure as granite bodies in the Himalaya in 10 million years (Le Fort et al., 1987).
To determine if the structures imaged on the 100-line were typical of the entire Himalayan range, MT data were collected in 2001 on additional profiles to the east and west (700-line and 800- line). When combined with MT data collected in India and Nepal, the results show that along strike variations in resistivity structure are minor. Thus it appears that the same tectonic processes are active along the entire Himalaya (Unsworth et al., 2005) and that the rift systems are relatively shallow features.
7. Magnetotelluric studies in Central and Northern Tibet
Passive seismic studies in Tibet have revealed that the deep seismic structure of northern Tibet is quite different to that of southern Tibet (Owens and Zandt, 1997). The mantle beneath southern Tibet has a relatively high seismic velocity, which implies that it is relatively cold. In contrast, the upper mantle beneath northern Tibet does not transmit shear waves, which implies that it is relatively hot, with asthenosphere at shallow depths. This observation provides additional support for local upwelling in the asthenosphere that may have followed from delamination of part of the lithosphere (Molnar et al., 1993). Other seismic tomography studies have imaged the Indian lithosphere descending vertically beneath central Tibet (Tilmann et al., 2003). Passive seismic data also give evidence for the southward subduction of Asian lithosphere beneath Northern Tibet (Kind et al., 2002).
To gain additional information on crust and upper mantle structure in these areas, the INDEPTH MT surveys were extended northward. MT data were collected on the 500-line in 1998. This crossed the Banggong-Nuijiang suture zone in Central Tibet. Logistics were managed by China National Petroleum Company and utilized oil exploration camps in the Lunpola Basin (Figure 5). No mature hydrocarbons have been found in this area, but the presence of intact sedimentary basins that have been uplifted more than 5 km, reveals that this uplift has occurred without breaking seals. Travel was particularly slow in this area and vehicles were frequently winched out of deep mud and rivers. In 1999 the 600-line was collected along the Lhasa-Golmud highway on the only hard top road into Tibet. These MT surveys showed that the mid-crustal conductor observed in Southern Tibet extended across the entire north south extent of the Tibetan Plateau and terminated at the Kunlun Fault (Wei et al., 2001; Unsworth et al., 2004). This layer acts as a mechanically weak zone that effectively decouples the upper and lower parts of the crust.
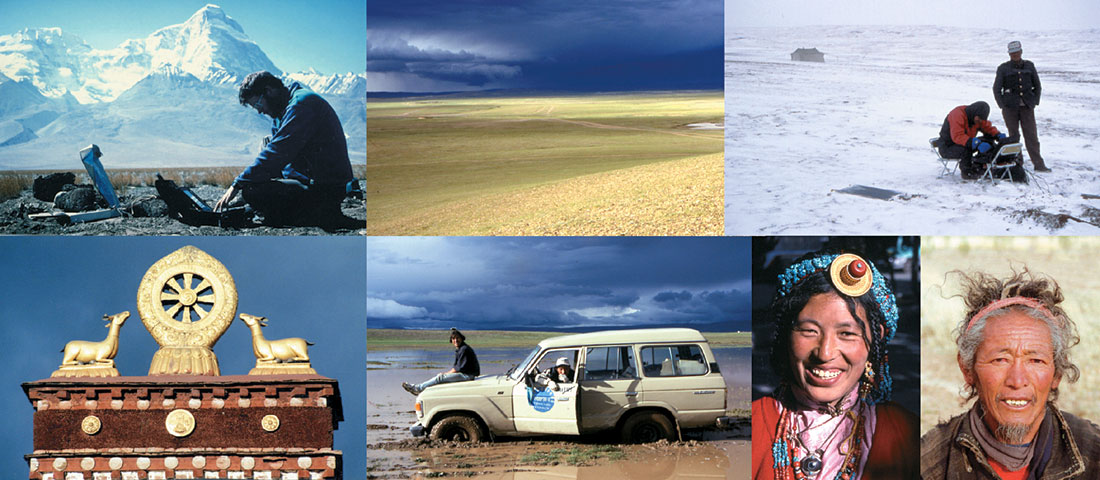
The presence of a major conductor in the mid-crusts reduces the sensitivity of MT to deeper structure. This is unfortunate since different geodynamic models make quite different predictions for lower crustal and upper mantle structure. In Southern Tibet a resistive lower crust and upper mantle appears to be present, but is not strongly required by the MT data (Spratt et al., 2005). In Northern Tibet, the low frequency MT data were of higher quality and suggest that the upper mantle has a low resistivity (Unsworth et al., 2004). This supports the idea of a shallow asthenosphere beneath Northern Tibet. Thus the mechanisms for plateau uplift are quite different in each area. In Southern Tibet, a thickened crust can support the 5 km elevation through an Airy type isotacy. In contrast, the Northern Tibetan plateau is supported by low density derived from elevated temperatures, in the Pratt type of isostasy. The remarkable fact is that there is very little expression at the surface of these fundamental changes at depths. It appears that regardless of the uplift mechanism, erosion and tectonic collapse will limit the development of topography above 5 km (Molnar et al., 1993).
The last decade has also seen the collection of high accuracy GPS measurements. Repeat measurements allow short term deformation rates to be determined. These show convergence rates of 40 mm/year between India and Eurasia, which decrease uniformly across the Tibetan Plateau (Zhang et al., 2004). These observations demonstrate that deformation is distributed and not localized in narrow zones between rigid blocks.
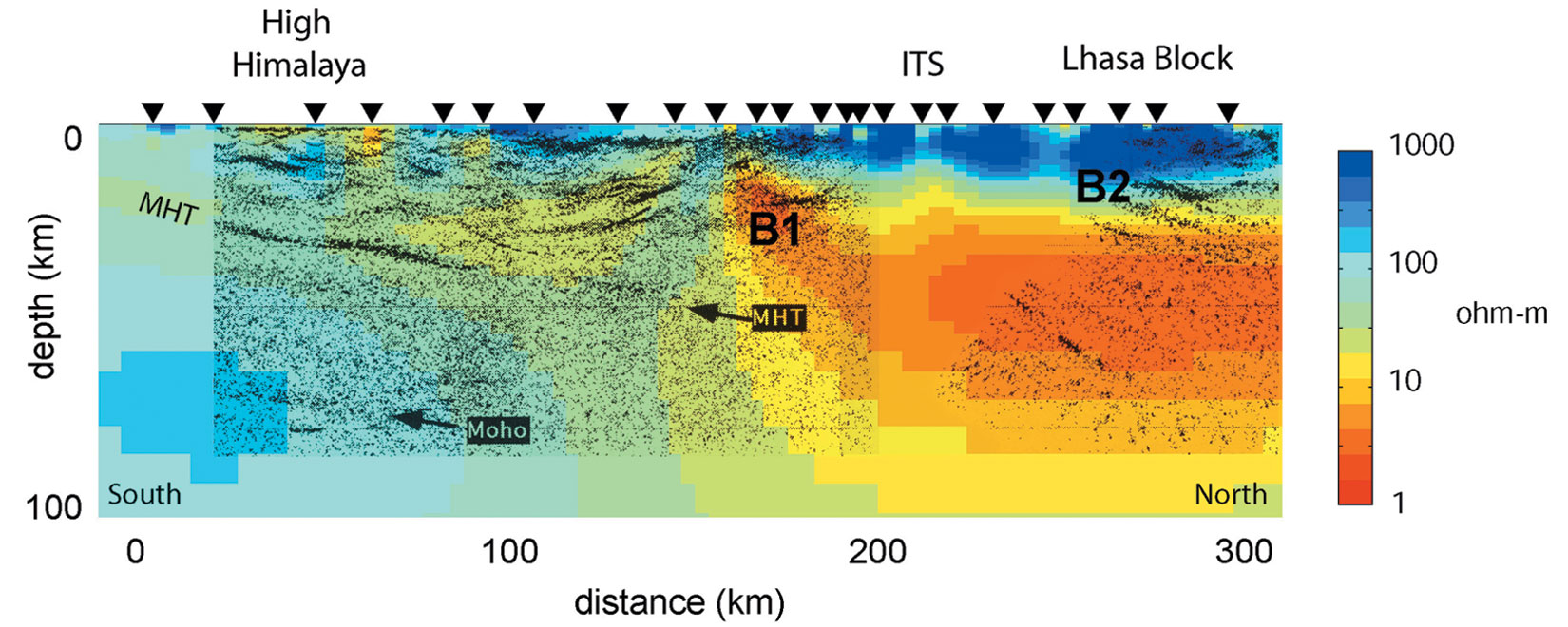
8. Continued growth of the Tibetan Plateau
In addition to motion of the surface, it has also been proposed that widespread crustal flow is occurring in eastern Tibet. Clark and Royden (2000) present a simple geodynamic model that elegantly explains why the margin of the plateau are steep in some areas and gradual in others. A channel of crustal flow requires a reduced viscosity and should have a low electrical resistivity. One end of channel was imaged by the MT profile in Northern Tibet (Unsworth et al., 2004) and MT data in Sichuan may be imaging the same feature (Jie et al., 2001). Growth of the plateau to the southeast occurs by active faulting and large scale crustal flow, perhaps with length scales in excess of 500 km.
The northern margin of the Tibetan Plateau represents a major boundary to plateau growth. Compressional forces are transmitted across the Tarim Basin to the Tien Shan where mountain building continues. The nature of the major strike-slip faults in Northern Tibet will be the focus of the final phase of the INDEPTH project, with combined seismic and MT fieldwork scheduled for 2007-8.
9. Summary and synthesis
The INDEPTH project has collected important geophysical and geological datasets across a large proportion of the Tibetan Plateau. Many of the key results are summarized in Figure 7. In Southern Tibet large scale thrust faulting has formed a crust that is in excess of 80 km thick. Crustal melting has occurred and when coupled with erosion, southward crustal flow in the Himalaya occurs. In contrast Northern Tibet has a slightly thinner crust that is underlain by a shallow asthenosphere. A mid-crustal zone of low resistivity is observed across the entire plateau. This is likely due to fluids and indicates a weak rheology. Thus deformation is characterized by distributed deformation and crustal flow, rather than the motion of rigid blocks. Thus the uplift of the Tibetan Plateau has originated from different mechanisms in different regions, while maintaining a uniform elevation from the Himalaya to the Kunlun Fault. Some of my Tibetan friends have an alternative explanation for why Tibet is so flat: the presence of yaks grazing for thousands of years has maintained the flat surface!
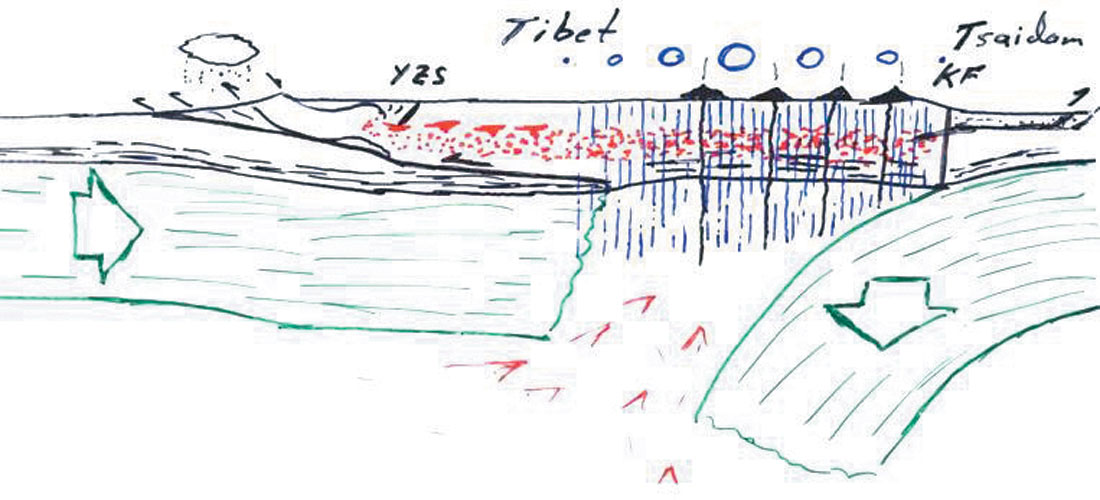
These studies of the India-Asia collision have revealed many first order details about continent-continent collisions. However, it is not clear from one example if other continent-continent collisions in the geological record have developed in the same way. The MT research group at the University of Alberta has recently begun a study of the Arabian-Asian collision zone in Eastern Turkey. MT data were collected in 2005 and results will be published in the near future.
Acknowledgements
The INDEPTH was funded by a number of international agencies, including National Science Foundation of the United States, and several funding agencies in China, Germany and Canada. This research was made possible by many people who worked in the field, in data analysis and in synthesis of the results. This article benefited from comments by Simon Klemperer.
Join the Conversation
Interested in starting, or contributing to a conversation about an article or issue of the RECORDER? Join our CSEG LinkedIn Group.
Share This Article